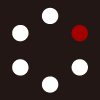
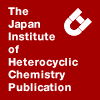
HETEROCYCLES
An International Journal for Reviews and Communications in Heterocyclic ChemistryWeb Edition ISSN: 1881-0942
Published online by The Japan Institute of Heterocyclic Chemistry
e-Journal
Full Text HTML
Received, 8th July, 2014, Accepted, 22nd September, 2014, Published online, 1st October, 2014.
DOI: 10.3987/REV-14-SR(K)3
■ Terpenoids Bearing the 7-Oxabicyclo[2.2.1]heptane (7-Oxanorbornane) Skeleton. Natural Sources, Biological Activities and Chemical Synthesis
Silvia Roscales and Joaquín Plumet*
Química Orgánica, Universidad Complutense de Madrid, Ciudad Universitaria, 28040 Madrid, Spain
Abstract
Compounds bearing the 7-oxabicyclo[2.2.1]heptane (7-oxanorbornane) subunits have been used extensively in the synthesis of a large array of complex organic structures. The ease of synthesis of compounds having this substructure in enantiomerically pure form, using Diels-Alder chemistry, is today an almost routinely experimental work. Moreover, the reactivity of these compounds, easily controllable and almost always associated to its ring-strain, makes them particularly valuable chiral building blocks. However, the presence of compounds showing this bicyclic skeleton in Nature has attracted less attention despite its relatively broad distribution. Many of these compounds have been shown to possess important biological activities presenting, in cases, unprecedented biogenetic origin. In addition, some of them have been considered as convenient synthetic targets enabling the development of new synthetic methodologies. In this article we have reviewed different families of terpenoids having in its structure this bicyclic subunit, paying particular attention to their natural sources, biosynthesis, biological activity and chemical synthesis.CONTENTS
1. Introduction. Scope of the review
2. Cantharidin and analogues
3. Monoterpenoids.1,4-Cineole and analogues
4, Oxanorbornyl-coumarins
5. Sesquiterpenoids
5-1. Epoxy-2,5-megastigma-6,8-dienes and related compounds
5-2. Chamigrene derivatives
5-3. Laurenditerpenol and derivatives
6. C-15 Acetogenines. Maneonene and Isomanoenene groups
7. Other sesquiterpenoids
8. Diterpenoids
8-1. The dactylomelane group
8-2. Diterpenoids with ent-clerodane (neo-clerodane) and dolastane skeleton
9. Triterpenoids
9-1. Cycloartane triterpenes
9-2. Baccharane triterpenes
9-3. Glycinoeclepins
10. Others terpenoid derivatives
11. Concluding Remarks
12. Acknowledgements
13. References and notes
1. INTRODUCTION. SCOPE OF THE REVIEW
Compounds derived from the 7-oxabicyclo[2.2.1]heptane (7-oxanorbornanes) 1 (Figure 1) are useful intermediates or starting material for the synthesis of more complex molecules including natural products and analogues also being valuable building blocks for polymers and material science.
The usefulness of this bicyclic system is consequence, at least, of two facts: first, because their syntheses –even in enantiomerically pure form- is relatively easy using, among other methods, Diels–Alder chemistry. Second, because the chiral information stored in the rigid skeleton may be transferred to their transformation products.
To the best of our knowledge, the more complete review on the chemistry of 7-oxanorbornane derivatives was published in 1999.1 Since then and to date a number of excellent reviews have been published, covering various specific aspects on the synthesis and synthetic applications of these compounds. An account of those, in our opinion more significant, is summarized in the following paragraphs:
1. Synthesis of cyclohexyl systems including hydroxycyclohexyl-β-amino acids and other cyclohexyl subunits, constitutive of a wide array of organic compounds. The synthetic methodology here described lies on the base- and acid-mediated fragmentation and transition-metal catalyzed ring-opening reactions of the oxygen-bridge of the oxanorbornene ring system.2
2. Synthesis of complex natural products starting from 7-oxanorbornene derivatives as well as the synthetic way to these building blocks.3 The utility of these transformations was highlighted in the enantioselective route to the central core 2 of banyaside and suomilide natural products (Scheme 1). Banyasides and suomilide are novel natural products belonging to the Aeruginosin family of serine protease inhibitors.4
3. Use of optically pure 7-oxanorbornene derivatives in the efficient synthesis of rare sugars,5 glycomimetics, polypropionates,5,6 anthracyclinone7 and aza-disaccharides.8
4. Synthetic applications of Ring-Rearrangement Metathesis (RRM) in 7-oxanorbornene derivatives and the related aza-analogues.9 Ring-rearrangement metathesis (RRM) refers to the combination of several metathetical transformations into a domino process, in which an endocyclic double bond of a cycloolefin reacts with an exocyclic alkene.
5. Synthesis of [6]n cyclacenes,10 the simplest subunits of carbon nanotubes. Cyclacenes has been defined as “Hoop-shaped systems composed of conjugated rings”. The necessary curved shape of the belt was achieved using the 7-oxanorbornene precursors 5 and 6 (Figure 2).
6. Synthesis of new functional polymers such as liquid crystals polyoxanorbornenes 8 from enantiomerically pure 7-oxanorbornenes 7 via Ring-Opening Metathesis Polymerization (ROMP) (Scheme 2).11
In addition, several 7-oxanorbornane derivatives are also found in Nature showing, in several cases, interesting biological activities. Only in reference 1 (see p. 13529-13546) this topic has been briefly mentioned and, on the best of our knowledge, no other review on this point can be found in the literature. In this account we intend to fill this gap considering this aspect under the points of views of the natural sources, biosynthesis, and biological activity of these compounds. In addition, the more recent chemical synthesis of some of them will be conveniently detailed.
We should indicate that our attention has focused on compounds bearing terpenic structure. Other related family of natural products such as carotene derivatives and some other natural products featuring the 7-oxanorbornene skeleton will not be considered here. The literature has covered until March 2014.
2. Cantharidin and analogues
Cantharidin 9 (2,6-dimethyl-4,10-dioxatricyclo[5.2.1.02,6]decane-3,5-dione, CTD, Figure 3)≠,12 was first
isolated in 1810 by Pierre Robiquet,¥,13 from Lytta vesicatoria (Spanish fly) sometimes incorrectly called Cantharis vesicatoria.
------------------------
≠Some cantharidin derivatives have been named as pseudocantharidins. Also norcantharidin 10 (Figure 3) has been designed as isocantharidin. This type of “nomenclature” will not be considered in this report. See reference 12.
¥Pierre-Jean Robiquet (1780-1840) is the author of important contributions in the field of natural products. For a summary of the contributions of Robiquet, see: J. Wisniak, Educacion Quimica, 2013, 24, 139.
This compound is the principle responsible for the aggressively vesicant properties of the coating of the eggs of the insect. Cantharidin shows potent antitumor activity and it seems to be the result of that CTD is a strong inhibitor of protein phosphatase type 1 (PP1) and type 2A (PP2A). Protein phosphatase PP2A, is an enzyme with broad substrate specificity and diverse cellular functions. Among the targets of PP2A are some proteins responsible of oncogenic signalling cascades. Protein phosphatase 1 (PP1) belongs to a certain class of phosphatases known as protein serine-threonine phosphatases. The PP1 has been found to be important, among others, in the control of cell division, apoptosis and protein synthesis.14 However, and due to its toxicity,15 the clinical application of this compound is limited.16 Diluted solutions of CTD can also be used as a topical medication in dermatology17 and their insecticidal activity18 and aphrodisiac properties19 have also been documented.
Norcantharidin 10 (NCTD, exo-7-oxabicylo-[2.2.1]heptane-2,3-dicarboxylic anhydride, Figure 3), the demethylated analog of CTD, causes fewer side effects than CTD and, like CTD, has been demonstrated as a potential agent against certain cancers.20 The NCTD is preferentially, but not always,21 toxic to cancer cells rather than normal cells, making this small molecule promising in cancer treatment.
Sodium salt of 7-oxabicyclo[2.2.1]heptane-2,3-dicarboxylic acid, 11 (Figure 3) also inhibit serine/threonine phsphatases.22 In particular is inhibitor of PP2B (calcineurin), a proteinphosphatase which is involved in a large variety of biological processes. The compound 11, designed in reference 12 as “desmethylcantharate” is a well-known synthetic herbicide named “endothall”. It should be pointed out that the compound “endothall” has been incorrectly formulated in reference 12 and in the reference 1a, p 13530.ϒ,23
Calcineurin is also known as protein phosphatase 3, and calcium-dependent serine-threonine phosphatase. Inhibition of calcineurin is associated with inmunosuppresion, and, in fact, calcineurin is inhibited by drugs such as cyclosporine.24
Organometallic complexes of 11 with Mn(II),25 Pt(IV)26 and La(III)27 have been reported that possesses important anticancer activity. On the other hand, metal complexes containing the subunit 11 and heterocyclic compounds showed interesting biological properties. That is the case of complex of [Ni(II)-2-aminomethylbenzimidazole],28 [rare earth-phenanthroline],29 [Co(II) and Zn (II)-2-amino- 1,2,3-thiadiazole],30 [Co(II) and Ni(II)-2-aminothiazole],31 [Co(II)-imidazole],32 [Mn(II), Ni(II) and Cu(II)-2-aminopyridine,33 [Cd(II)-2,2-bipyridine and phenanthroline],34 [transition metals-2-amino- benzothiazole],35 and [Mn(II), Ni(II) and Zn(II)-2,2’-bipyridine].36
Although cantharidin never seems to have been isolated from plant sources, the demethyl derivative palasonin 12 (3-methyl-7-oxabicyclo[2.2.1]heptane-2,3-dicarboxyxlic anhydride), which lacks one of the angular methyl groups of cantharidin, (Figure 3), was initially isolated from the seeds of the Indian tree, Butea frondosa (Leguminoseae).37 The anthelmintic properties of 12 are well documented.38
Cantharimides are compounds in which the anhydride oxygen atom of cantharidine has been replaced by a N-substituted derivative. From the Chinese beetle Mylabris phalerata PALLAS,ξ cantharimides 13-15 have been isolated.39 In these compounds the N-substituents are L-lysine, L-ornithine and L-arginine moieties respectively. Later and from the same insect five cantharimide-derivatives 16-20 were also isolated.40 Three of them were cantharimide dimers (compounds 18-20) which consists of two units of cantharimide combined with a tri-, tetra- and penta-methylene group. Other simple cantharimides have been previously isolated from natural sources. It is the case of the N-phenyl and N-hydroxyimides 21 and 22. Compound 21 was isolated from Butea monosperma41 whereas compound 22 is an ingredient of Mylabris phalerata42 (Figure 4).
Before the isolation of compounds 13-20 several cantharimide derivatives were rationally designed,43 synthesized and evaluated as inhibitors of PP1 and PP12A.44 More recently45 thirty-two cantharimides containing aryl, pyridyl, azolyl, thiadiazolyl, diaminophenyl, aminosulfanyl, sulfamethoxazolyl, or sulfadiazine moieties were synthesized by heating cantharidine at 200°C in toluene and Et3N along with the corresponding amines. All compounds were tested for their capability to suppress growth of several human carcinoma cell lines, concluding that the cytotoxic effects were produced by many different structural features of the cantharidinimide derivatives. For instance, the in vitro results suggested that decreasing electron negativity on the substituent-donating group might increase their cytotoxicity.
--------------------------------------------
ϒThe names assigned to endothall constitute an authentic puzzle. Trade names for the acid form of endothall include Aquathol, Hydrothal-47 and Hydrothal-191. Trade names for the disodium salt of endothall include Accelerate, Des-I-Cate, and Niagrathol among others. See: “The Agrochemicals Handbook”, Third Edition. Royal Society of Chemistry Information Systems, Surrey, England, 1994.
ξThis insect has been used in traditional Chinese medicine for the treatment of cancer. See: “The Dictionary of Chinese Drugs”. Shanghai Science and Technological Publishers, Tokyo, 1985, Vol. III, pp. 2196-2198.
Eighteen biologically active cantharidinimines with general structures 23-25 (Scheme 3) were prepared by heating cantharidin with the appropriate azolamines, sulfanils, and pyridinamines in ethanol.46 The cytotoxic effects of these cantharidinimines seemed to be better than others cantharimides previously synthesised.
The mechanism of the biosynthesis of cantharidin is not exactly known although appears to be clear that it implicates some unprecedented transformations of farnesol.47
From 1953,48 different synthetic approaches to cantharidin have been carried out using Diels-Alder chemistry. Among them, the synthesis of Stork,49 Schenk50 and, more recently, Dauben (using high-pressure conditions)51 and Grieco (LiClO4-promoted Diels-Alder cycloaddition)52 should be emphasized. These synthetic efforts as well as the chemical transformations of cantharidin in others related, biologically active compounds, have been recently summarized in the appropriate reviews. 53 Nevertheless, some recent, significant aspects of this chemistry applied to the synthesis of cantharidin analogs are highlighted as follows.
The 7-S analogues of cantharidin derivatives 26-28 were synthesized in acceptable yields (ca 60%) using high-pressure Diels-Alder reaction of tiophen with dimethyl maleate, maleic anhydride and maleimide respectively≠ (Scheme 4).54
-----------------------------------------
≠ Interestingly compound 28 was found to be >30-fold selective than 27 vs. PP2A. See reference 54.
High-pressure synthesis of compounds 26 and 27 has been previously reported in ca 40% isolated yields. 35 Also compound 26 was synthesized in LiClO4-Et2O mixtures albeit in lower yield.54
Optically pure norcantharidin derivatives 29-32 were synthesized56 by reaction of (R)-and (S)-2- benzyloxyethyl)furan 33 and 34 respectively and maleic anhydride followed by hydrogenation (Scheme 5).
Compounds 33 and 34 were prepared by reaction of (R)- and (S)-1-(2 furyl)-ethanol, respectively, with benzoyl chloride (Et3N, DMAP, CH2Cl2). Interestingly it was observed that (1R)-isomers 30 and 32 inhibit PP1 and PP2A whereas the (1S)-isomers 29 and 31 have negligible inhibitory activity for PP1 and PP2A.
Racemic palasonin 12 was synthesized57 from furan and methoxycarbonyl maleic anhydride 35 using the lineal, nine-step sequence (6% overall yield) depicted in Scheme 6.
Two comments appear to be opportune regarding this synthetic approach: firstly, the concomitant reduction of the resulting adduct of the Diels-Alder reaction of 35 and furan to give anhydride 36 under the reaction conditions i). However, the reaction of citraconic anhydride (3-methylmaleic anhydride) 44 (see Scheme 7) with an excess of furan under hydrogenation conditions afforded only methylsuccinic anhydride.58 On the other hand, direct transformation of 41 into 12 appears to be an appealing possibility. Nevertheless, all attempts to achieve this transformation were unsuccessful mainly due to the vigorous conditions necessary to hydrolyze the N-phenylimide ring. In contrast, the nitrophenylimides 42 and 43 were easily hydrolyzed to give 12. The purification of 12 was achieved by preparative GC.
In the same paper, Dauben et al.58 reported a two-step synthesis of racemic 12 (92% overall yield) by high-pressure Diels-Alder reaction of furan and citraconic anhydride 44 followed by catalytic hydrogenation (Scheme 7).
It should be pointed out that, under pseudo-first order conditions (furan as solvent), adduct 45 was obtained in 1.7% yield (at 20 ºC) and 1.5% yield (at 40 ºC). The values of the equilibrium constant at both temperatures were determined as K= 1.1x10-3M-1 (20 ºC) and K= 8x10-4 M-1 (40 ºC). Other kinetic parameters are: k1= 1.1x10-9 M-1s-1 (20 ºC) and k1= 4.7x10-8M-1s -1 (40 ºC); k1φ = 3.7x10-6 s-1 (t1/2 = 52 h, 20 ºC); k1φ = 5.9x10-5 s-1 (t1/2 = 3.3 h, 40 ºC).
On the other hand, and under catalysis by 5.0M LiClO4-Et2O (Grieco’s conditions), the reaction of 44 and furan gave less than 10% yield of 45 with K= 1.0x10-2M-1 at rt.58
Racemic palasonin was resolved by reaction with (S)-2-methylbenzylamine followed by separation of the diastereomeric amides, saponification to the diacids and further reaction of the individual diacids with thionyl chloride. The combination of a pyrazole ring≠,59 fused to the norcantharidin framework in one single structure such as 46 (Scheme 8) constitutes an appealing possibility in order to improve the antiproliferative activities of norcantharidin derivatives. Also the introduction of a phosphate group should improve the aqueous solubility of these compounds. with in situ-generated diarylnitrilimines.ξ,60
------------------------
≠Although pyrazoles are rarely found in nature probably due to difficulty in the formation of N-N bond by living organisms, they exhibit numerous biological activities. For recent reviews, see reference 59.
ξCatalytic oxidation of aldehyde hydrazones 50 with chloramine T leads to the formation of nitrilimines 51. See reference 60.
Cell-growth inhibition assay shows that compounds 46b and 46c where even more potent than cantharidin with aqueous solubility greatly improved.
3. Monoterpenoids. The 1,4-cineole and analogues
The cyclic monoterpene 1,4-cineole (1-isopropyl-4-methyl-7-oxabicyclo[2.2.1]heptane 52 (Figure 5), first described in 1907,61 is widely distributed in nature. Their use as fragrance and flavoring agent is well documented. For instance, compound 52 is a major component of lime (Citrus aurantifolia) and eucalyptus (Eucalyptus polybractea) being also present in apricot (Prunus armoniaca), orange juice (Citrus sinensis L. Osbeck), grapefruit juice (Blettaria cardamomum Matum), laurel (Laurus nobilis L.) and rosemary (Rosmarinus officinalis L.).62
The biosynthesis of 1,4-cineole 52 (Scheme 9) has been determined by feeding to plants with radioactively labeled acetate and subsequent determination of the labeling pattern in the terpene (Scheme 9).63 Thus, transformation of geranyl diphosphate 53 into α-terpineol 59, via α-terpinyl cation 58,64 followed by a sequence hydration-dehydration of the intermediates 1,8-terpin 61, γ-terpineol 62, and 1,4-terpin 63, afforded finally 52. It should be indicated that the isomerization of α-terpineol 59 to 1,8-cineole 60 and 1,4-cineole 52, catalyzed by H3PW12O40 in homogeneous and heterogeneous systems, has been reported.65
On the other hand, the α-terpinyl cation 58 can undergo a range of cyclizations, rearrangements, and hydride shifts which conforms the biogenetic way for most of the cyclic monoterpenes such as camphene 64, α− and β-pinene (65 and 66) fenchol 67, terpinolene 68, limonene 69, car-3-ene 70, β-phellandrene 71, γ-terpinene 72 and sabinene 73 besides the already mentioned α-terpineol 59. Moreover, the geranyl 54 and linalyl 57 cations also are precursors of the acyclic monoterpenes geraniol 74, linalool 75, myrcene 76 and (E)-β-ocymene 77 (Figure 6).66
The α-terpineol 59 is also the biogenetic precursor of 1,8-cineole (1,3,3-trimethyl-2-oxabicyclo[2.2.2]octane, eucalyptol) 60, an isomer of 1,4-cineole (Figure 5). The use of 1,8-cineole as flavoring, fragrance and cosmetic,66 insecticide67 and repellent68 is well known. In contrast, eucalyptol is attractive to males of various species of orchid bees.69 Compound 60 was also found to control airway mocus hypersecretion and asthma70 and inhibit cytokine≠,71 production in cultured human limphocytes and monocytes.72 This compound was found effective in the treatment for non-purulent rhinosinusitis with minimal side effects.73 Reduction of inflammation and pain when applied topically74 and activity against leukemia cells in some cultured leukemia human cells has also been reported.75
As a consequence of their applications as flavoring agent in alimentation and cosmetic, the metabolism of 52 has been considered.76 Although there are not in vivo studies in humans, after oral applications to rabbits, rats and human liver microsomes, the following metabolites have been identified (Scheme 10): 3,8-dihydroxy-1,4-cineole 78, 8,9-dihydroxy-1,4-cineole 79, 1,4-cineole-8-en-9-ol 80, 9-hydroxy-1,4- cineole 81, 1,4-cineole-9-carboxylic acid 82 and 2-exo-hydroxy-1,4-cineole 83. Probably, 3-hydroxy-1,4-cineole 84 is the metabolic precursor of 78 and 79.
--------------------------------
≠Cytokines constitute a category of small proteins that are important in cell signalling, being produced by broad range of cells, including immune cells as well as endothelial cells, fibroblasts, and various stromal cells. They are important in connection with some diseases and, specifically, in host responses to infection, immune responses, inflammation, trauma, sepsis, cancer, and reproduction. See reference 73.
The 1,4-cineole 52 possesses important herbicidal activity.77 In this context the 2-hydroxy derivative (exo-2-hydroxy-1,4-cineole) 83, a constituent of the essential oil of Ferula jaeschikeana 78 is of interest as starting material for the synthesis of the 2’-methylbenzyl ether derivative 85, a commercial herbicide known as cinmethylin (see Figure 7, see below).≠
The microbial oxidation of 1,4-cineole 52 was considered using different microorganism (Scheme 11). For instance, Streptomyces griseus yielded 8-hydroxy-1,4-cineole 81 as the major hydroxylation product together 83 and 86.79 Bacillus cereus and Penicillium frequentatis yield changing ratios of 83 and 86.80 In the case of P. frequentatis, both enantiomers of ketone 87 were also produced. Using Aspergillus niger, compound 86 was established as the major product.81 In addition, different oxidases present in plant cultured cells of Catharantus roseus produced mixtures of secondary and tertiary alcohols.82
The chemo- and stereoselectivity of this microbial oxidation has been studied in deep using Bacillus cereus and Streptomyces griseus as biocatalysts. The hydroxylation with B. cereus displays high face stereoselectivity yielding only 2(R)-exo-hydroxy-1,4-cineole 83 whereas S. griseus gives 8-hydroxy-1,4-cineole 81 as major product together enantiomeric mixtures of 83 and 86 (Scheme 11).83
It was hypothesized84 that the mode of action of 1,4-cineole derivatives as herbicides consists in blocking the conversion of tyrosine to 4-hydroxyphenylpyruvate (4-HPP). This transformation is catalyzed by tyrosine aminotransferase.ξ,85 In the prenylquinone pathway, tyrosine aminotransferase provides plastoquinoneϒ,86 a cofactor of phytoene desaturase, an oxidoreductase involved in carotenoid biosynthesis.87
On the other hand, 1,4-cineole and the metabolic conversion product of the synthetic herbicide cinmethylin, are potent inhibitors88 of asparagine synthetase, the key enzyme in asparagine biosynthesis.≠ Asparagine synthetase plays an important role in nitrogen mobilization.89 The biological activities of 1,4-cineole as anxiolytic,90 antimicrobial91 and permeation agent for percutaneous absorption of drugs92 have also been reported.
Related with 1,4-cineole is the eight-carbon system rengyoxide 89 (Figure 7), originally isolated from Forsythia suspensa fruits93 and more recently from the wastewaters of 'Megaritiki', 94 an olive cultivar widely used in Greece for the production of low polyphenol olive oil and table olives.
Finally it should be indicated that, in reference 1 p. 13531, the structure 90 was attributed to mullilam diol, isolated from Zanthoxylum rhetsa, a plant which show antibiotic activity and prescribed in dyspepsia and diarrhoea. This structure has been revised and conclusively verified to possess a structure, (±)-p-menthan-1α,2β,4β-triol 91 (Figure 7).95
--------------------------------------------
ξFor detailed studies on the role of tyrosine aminotransferase in plants see reference 85.
ϒCompounds with structure of 2,3-dimethyl-1,4-benzoquinone showing isoprenyl side chains of different length in position 5 (compounds with structure 88) are designated as plastoquinone-n (PQ-n) where n varying from 6 to 9.
≠Asparagine synthetase catalyzes the synthesis of asparagine from glutamine and aspartate through an amidotransferase reaction
4. OXANORBORNYL-COUMARINS
Farnesiferol C 92 (Figure 8) has been isolated from species of the genus Ferula such as F. assafoetida,96 F. szowitsiana97 and F. sinkiagensis.98 Compound 92 show antiviral,99 antiangiogenesis100 and anticancer activity.
Several biogenetic-type syntheses of 92 by acid-catalyzed cyclizations of epoxy polyenes≠,101 have been reported. For instance, acid-catalyzed cyclizations102 of trans, trans umbelliprepin oxide 93, easily prepared from the natural coumarin umbelliprepin,ϒ,103 94, afforded 92 albeit in low yield (Scheme 12).
-----------------------------------------------------------
≠The acid-catalyzed cyclization of epoxi polyenes has attracted much attention due to the discovery that analogous enzyme-catalyzed reaction are involved in the biosynthesis of a large array of terpenoids. See reference 101
ϒCompound 94 was isolated from Ammi majus L. fruits. See reference 103.
Other convenient biogenetic-type synthesis of 92 was carried out from 10,11-epoxyfarnesyl acetate 95104 using a key step a zeolite105 NaY-catalyzed cyclizations to give 96. Further standard transformations of 96 into bromide 97 and final coupling of 97 with umbelliferone 98 yielded 92 (Scheme 13).106 Attempts to perform the direct transformation of 93 into 92 using zeolite NaY were unsuccessful.
A non biogenetic-type synthesis of racemic 92 together the Z-isomer 104 has also been reported (Scheme 14).107 The key step was the Baeyer-Villiger oxidation of decalone 99≠ to give lactone 100 which rearranges to the 7-oxanorbornane derivative 101. Standard Barbier-Wieland degradation of this compound to 102 followed by ketone formation and vinylmagnesium bromide addition gave 103. Further sequence of bromination and coupling with 98 afforded a mixture of 92 and 104 in ratio 3:1.
-------------------------------------------
≠Compound 99 was synthesized by Robinson annelation of 2-methyl-cyclohexanedione with ethylvinyl ketone and further elaboration of the resulting product. See: D. L. Snitman, M. Y. Tsai, and D. S. Watt, J. Org. Chem., 1979, 44, 2838.
The optical antipode of the natural product, (+)-farnesiferol C 105 was synthesized from the magnesium salt of the phenylglycinol derivative 106 by means of an intramolecular furan Diels-Alder (IMDAF) reaction. (Scheme 15).108 In this way, a mixture of compounds 107 and 108 (ratio 107:108 = 78:12) was obtained. From 107, a 15 step sequence afforded 109 which, by formation of the bromide and reaction with the potassium salt of 98, gave 105.
Creticacoumarin 111, an oxidized form of 92 (Figure 9)109 was isolated from Anthemis cretica. On the other hand, the natural coumarin (-)-3’, 6’-epoxyauraptene was first isolated110 from various Aster species (genus of flowering plants of the family of Asteraceae) and its structure determined as 112 (Figure 9) both chemically111 and by X-ray analysis.112
The biomimetic synthesis of 112 has been carried out starting from the 7-geranyloxycoumarin 113 (auraptene) which, in turn, was synthesized from geraniol 74 via geranyl bromide 115 and further reaction with the sodium salt of umbelliferone 98 (Scheme 16).113
In the last decade auraptene was seen to exert important biological activivities such as anticancer, anti-bacterial, anti-protozoal, anti-fungal, anti-inflammatory and anti-oxidant.114
The transformation of 113 into 112 was carried out by way of the terminal epoxide 114, also isolated from the same natural source.112
Epoxide 114 has been synthesized in racemic form via a sequence hydroxylation-hypobromination115 or in optically pure form using Sharpless asymmetric epoxidation116 or microbial dihydroxylation.117 Treatment of 114 with Lewis acid, in particular SnCl4, afforded 112 (Scheme 17). The best yield reported was 60%.113
The racemic 2-epimer of 112, compound 116, has been synthesized from ketone 117≠,118,119 easily accessible by Diels-Alder cycloaddition of 2-methylfuran 118 and 2-chloroacrylonitrile 119 (Scheme 18).120 Double methylation of 117 at C-3 followed by Wittig olefination gave 120 which, by oxidative hydroboration followed by mesylation and reaction with the potassium salt of 98, gave 116.
----------------------------------------
≠The use of ketone 117 in the synthesis of natural products containing the 1,3,3-trimethyl-7-oxabiyclo[2.2.1]heptane moiety has been reviewed. See reference 118. On the other hand, compound 117 serves as versatile template for the synthesis of other subunits of natural products and analogues. See reference 119.
5. SESQUITERPENOIDS
5.1 . Epoxy-2,5-megastigma-6,8-dienes and related compounds.
These compounds, as 6(E),8(E)-, 122 and 6(E)-8(Z)-, 123 diatereoisomers (Figure 10) were first isolated121 from Osmanthus absolute and their structures determined by synthesis from ethyl α-safranate (see below). Both compounds have been also detected as aroma component of Malphigia glabra.122 The 6(E),8(E) diastereoisomer 122 was detected123 in the essential oils of Centaurea sericeae and Centaurea ensiformis and in the volatile flavor of Malpighia emarginata.124 The basic structure, but with undetermined stereochemistry, has been also detected as volatile component of honey bush (Cyclopia subternata). 125
The first synthesis of compounds 122 and 123 was achieved starting from ethyl α-safranate 124 (Scheme 19).121,ϒ
--------------------------------------
≠The use of ketone 117 in the synthesis of natural products containing the 1,3,3-trimethyl-7-oxabiyclo[2.2.1]heptane moiety has been reviewed. See reference 118. On the other hand, compound 117 serves as versatile template for the synthesis of other subunits of natural products and analogues. See reference 119.
ϒInterestingly, this synthetic sequence, reproduced by Mori et al., confirms that the natural, minor compound 123 (see reference 121) was isolated in only 11% e.e. See : K. Mori and H. Tamura, Tetrahedron, 1986, 42, 2643.
This compound was transformed into 2-hydroxy-α-cyclocitral 125 through a five-steps sequence, including sensitized photooxidation, catalytic hydrogenation, dehydration, reduction with litium aluminium hydride and re-oxidation with pridinium chlorochromate. The base-catalyzed condensation of 125 with acetone afforded a mixture of compounds 126, 127 and 128, this later probably arising from intramolecular Michael addition of 126. After purification, the hydroxyketones 126 and 127 were transformed into diol 129. Acid treatment of 129 gave a mixture of 122 and 123 in ratio 85:15 and 50% overall yield in this last step.
A convenient synthesis of 122 has been carried out126 from ketone 117. This compound was transformed into ketone 130 which, after reaction with the Grignard reagent derived from (E)-1-bromo-2-butene and dehydration of the resulting product 131 via phenyluretane 132, afforded 122 (Scheme 20).
Alcohol 133 (Figure 11) has been found as trace component in Osmanthus absolute and can be also obtained (10%) in the acidic treatment of compound 129.121 On the other hand, alcohol 134 (Figure 11) was found as component of essential oil of Lank127 and Japanese Saifu tobacco128 and has been also isolated from Saussurea cordifolia.129 This compound constitutes the aglycon (C-9-O-β-D)-of the glucosyde Crotanoloside C, from Crotalaria zanzibarica.130
The synthesis and structure confirmation by X-ray analysis of compound 134 has been accomplished131 starting from dehydroionone derivative 135132 (Scheme 21).
5.2. Chamigrene derivatives.
Compounds 143 (oxachamigrene) and 144 were isolated from Laurentia obtuse.133 Two others halogenated sesquiterpenoids, compounds 145 and 146, were also isolated from L. obtuse134 and L. pannosa135 respectively (Figure 12).≠
--------------------------------
≠For an exhaustive review on the halogenated organic molecules of Rhodomelaceae origin, see: B. G. Wang, J. B. Gloer, N. J. Ji, and J. C. Zhao, Chem. Rev., 2013, 113, 3632. The Rhodomelaceae is the largest marine red algal family. A total of 1058 naturally occurring compounds were isolated and characterized from Rhodomelaceae (1960-early 2012). Of these, 76% were halogenated molecules.
Compounds 143 and 144 show the chamigrene (spiro [5,5]undecane) skeleton 147, formally originate from linking the bonds C1-C6 and C6-C11 of farnesane 148 (Scheme 22a) whereas compound 145 representing a new class of cyclohexylcyclohexane-containing carbon skeleton 149 that may be rationalized as arising from C11−C14 cyclization of the bisabolane framework 150 (Scheme 22b). Finally, compound 146 (pannosane) possessing a new carbon skeleton 151 appears to result from a methyl migration from C11 to C10 of chamigrene framework 147 (Scheme 22c).136 For compounds 143, 144 and 146 two biogenetic pathways have been proposed starting from γ-bisabolene 152. Both sequences are outlined in Schemes 23 and 24.
5.3. Laurenditerpenol and derivatives.
(-)-Laurenditerpenol 166 (Figure 13), isolated from the red alga Laurencia intrincata,137 was shown to inhibit activation of hypoxia inducible factor 1 (HIF-1). The HIF-1 plays an important role in cellular and systemic responses to hypoxia (condition in which the body or a region of the body is deprived of adequate oxygen supply). This inhibitory activity constitutes a valuable alternative for the late-stage cancer therapy.138
Considering that brominated terpenes are among the most common natural products produced by Laurencia spp. seems logical to assume that the compound 166 may be biogenetically formed from farnesyl pyrophosphate 167 through a sequence bromine assisted-cyclization-debromination of a diterpene precursor 171 as indicate in Scheme 25.137
A synthesis of racemic 166 and its diastereomer 175 was achieved139 in a convergent fashion (Scheme 26) by coupling fragments 176 and 177 via Julia olefination procedure followed by reduction and deprotection of the dehydro derivatives 180 and 181. Aldehyde 176 was synthesized from enone 178140 in a nine-steps sequence whereas sulfone 177 was prepared from the DA adduct of 2,5-dimethylfuran and maleic anhydride141 179 in eight steps. Coupling of 176 and 177 yielded a mixture of cis-and trans- products 180-183 from which the desired E-products could be separated by flash chromatography and submitted to diimide reduction to give a 1:1 mixture of 184 and 185.
Treatment of this mixture with TBAF afforded 166 and 175 which were partially separated by chromatography. In the context of the synthetic sequence depicted in Scheme 26, two comments are pertinent: a) Unfortunately the Julia-Kocienski olefination between lactol 203, prepared from 188 by reduction with DIBAL, and sulfone 177 failed probably because the lactol exits mainly in the cyclic form (Figure 14); b) Compound 188 is known as wine lactone. Wine lactone was found in white wines. There are 8 possible isomers of wine lactone being the (3S, 3a S, 7aR) isomer the only one that has been found in wine. The odour threshold of the wine lactone is 0.00001-0.00004 ng/l in air.142
Compounds 187 and 192 have been synthesized143,144 in 99%ee and 87%ee respectively according with the following protocols (Scheme 27): starting from the DA adduct of 1,3-cyclohexadiene and methyl acrylate 195,≠ the nitrosobenzene-mediated transformation into ketone 196 followed by Baeyer-Villiger oxidation, ring-opening reaction and MeMgBr addition, cleany afforded 187 without any loss of optical purity (Scheme 27a). On the other hand, the synthesis of 192 was achieved by Diels-Alder reaction of 2,5-dimethylfuran and allenic ester 198 in the presence of catalyst (S)-201, followed by reduction and catalytic hydrogenation in the presence of Willkinson’s catalyst (Scheme 27b). Thus, this procedure constitutes a convenient enantioselective approach to (-)-166.
-----------------------------------
≠Compound 195 has been previously synthesized by DA reaction of 1,3-cyclohexadiene and methyl acrylate (90% yield, 99%ee) using the chiral oxazaboralidinone triflate 201 as catalyst. See Scheme 27 and reference 143. On the other hand, the catalytic DA reaction of 198 has also been carried out in the presence of catalyst (S)-202 using cyclopentadiene and furan as diene components and several acrylic esters as dienophilic counterparts. In most cases, (S)-202 was found to be superior to catalyst (S)-201. See reference 144.
A related approach to (-)-166 has been accomplished145 through the addition of the organolithium compound derived from 204 to aldehyde 176 (see Scheme 26). The oxanorbornane derivative 204 was prepared from 192 which was in turn synthesized in seven steps from 205 (Scheme 28a). This compound had been previously prepared by catalytic enantioselective Diels-Alder reaction of diethyl fumarate and 2,5-dimethylfuran146 followed by hydrogenation of the resulting cycloadduct.
On the other hand, aldehyde 176 was synthesized in this approach from compound 213 (eight steps, Scheme 28b).
Compound 213 was obtained in three steps as a 1:1 mixture of diastereomers from S-limonene 212.147 Lithiation of 204 followed by slow addition of a solution of 176 in diethyl ether led to the formation of alcohols 217 in 30% yield as a 3:1 mixture of C(8) epimers. After a further three-steps sequence, compound (-)-166 was obtained in 31% yield over the last four steps (Scheme 28c).
It should be pointed out that the mixture of epimeric lactones 188 and 215 arises from the non isolated compound 221 (Figure 15), via allylic rearrangement and lactonization.
The absolute stereochemistry of 166 was determined through an asymmetric total synthesis as well as their distereomers 222-226.148 The synthetic scheme was based on the coupling of the optically pure sulfone (-)-227 and the halide 228 which were in turn prepared from 2,5-dimethyl-3(2H)-furanone 229 and R-(+)-pulegone 230 respectively. The preparation of compounds 227and 228 are outlined in Scheme 29. Reaction of 229 with methyl crotonate 231 produced racemic 232ξ, 149 which, after thioketalization and hydrolysis afforded racemic 233. Resolution of this compound via N-acyloxazolidinones (-) and (+)-234 followed by standard transformations gave pure sulfones (-)-227 and (+)-227 (Scheme 29a). The synthesis of both sulfones (-)-227 and (+)-227 were carried out starting from (-)-234 and (+)-234 respectively, through the same reaction sequence. Only the stereostructure of the “natural” moiety [from (-)-234] is showed. The crystal structure of (-)-234 established the absolute configuration of the oxanorbornene ring system of 166, previously proposed on the basis of NMR experiments.
-------------------------------------------------
ξ The formation of 232 may be due to either an “anion-assisted” DA cycloaddition through intermediate 254 or a sequential Michael additions. See reference 149.
The coupling of (-)-227 and bromide 228 afforded the sulfone 236 which was transformed into alcohol 237 in a stereo-and regioselective fashion via a sequence hydroboration-oxidation. The stereochemistry of this reaction may be explained in terms of electrophilic borane addition according with the Felkin-Anh-Houk hypothesis.150 Further transformation of 237 into ketone 238 was accomplished through a six-step sequence. Regioselective reduction of 238 gave a chromatographically separable C1 epimers 222 and 223 (Scheme 30a).
The remaining stereoisomers 166 and 224-226 were synthesized from sulfone 236 following the previously developed synthetic strategy but altering the reaction sequence (Scheme 30b). Thus, considering that the observed stereoinduction in the hydroboration reaction of 236 was consequence of the presence of the phenylsulfone functionality, compound 236 was first desulfonylated. After reduction and further sulfinylation, a chromatographically separable mixture of diastereomers 241 was obtained.
Separation of both C7 epimers, reductive elimination of phenyl sulfide in 241 and deketalization yielded ketone 242 which, after epimerization to 243, dehydrogenation to enone 244 and regioselective reduction, produced stereoisomers 224 and 225 in ratio 224:225 = 2:3.
Following the same synthetic sequence, stereoisomers 166 and 226 were also synthesized from 241b (Scheme 30c). Synthetic 166 shows 1H- and 13C-NMR spectra, rotation value and TLC behaviour identical to a sample of the natural product.
Compounds 223 and 224 were evaluated for their ability to inhibit hypoxia-induced HFI activation, showing that the inversion of configuration at C7 (compound 224) was associated with a 76% drop in potency relative to synthetic 166 whereas inversion of configuration at C1, C6 and C7 (compound 223) results in total loss of biological activity.
A notable improvement of this synthetic sequence applied to the total synthesis of both enantiomers of laurenditerpenol has been achieved151 by modification of the following original features:
* Bromide 228 was substituted by bromide (+)-245. This compound was synthesized in four steps from (S)-citronellal 246 via ZnCl2-catalyzed intramolecular Prins cyclizations152 to give (+)-isopulegol 247 following by silyl protection and regioselective allylic bromination (Scheme 31). In the synthesis of (+)-247 from 246, minor amounts (11%) of (-)-neo-isopulegol 255 were also obtained. The mixture of (+)-247 and (-)-255 was easily purified by SiO2 column chromatography.
* Coupling of (+)-245 with sulfone (-)-227148 (Scheme 32) gave (+)-248 which, after TBS deprotection, afforded 249. Hydroxy-directed hydrogenation≠,153 of this compound using Crabtree’s catalyst154 yielded (-)-250 which was desulfonylated to (-)-251. The transformation of (-)-251 into (-)-166 was achieved via the ketone (-)-252 and enone (-)-253 using the same synthetic protocols depicted in Scheme 30. The unnatural (+)-166 was synthesized following the same synthetic sequence starting from sulfone (+)-227148 and bromide (-)-245 prepared from commercially available (-)-isopulegol.
--------------------------------------
≠The stereochemistry of this directed-hydrogenation reaction appears to be a consequence of simultaneous coordination of Ir to the hydroxyl group at C1 and the alkene between C7-C19, forming a rigid, chair-like intermediate. In this context, see reference 153.
6. C -15 ACETOGENINS. MANOENENE AND ISOMANOENENE GROUP
The C-15 acetogeninsϒ,155 belong to a large family of halogenatedξ compounds commonly found in species of the family Rhodomelaceae. The manoene group features a skeleton of hexahydro-2,6-methanofuro[3,2-b]furan 256 whereas the biscarbocyclic skeleton of isomaneonenes consist in a system of octahydro-1,3-dioxa-2,4-mathanocyclopenta[cd]pentalene 257 (Figure 16).
-------------------------------------------------
ϒThe difference between C-15 acetogenins and sesquiterpenes consists in their different biogenetic pathway. The sesquiterpenes, like all terpenoids, are formed by condensation of isopentenyl pyrophosphate (see reference 64) whereas C-15 acetogenins arise biogenetically from a common C-15 precursor which derives from a C 16 fatty acid. See reference 155.
ξIn these halogenated compounds as well as in halogenated sesquiterpenes the role of marine haloperoxidases appears to be well documented: A. Butler and J. N. Carter-Franklyn, Nat. Prod. Rep., 2004, 21, 180.
All manoenene derivatives isolated up the date are quoted in Table 1. All of them have been isolated from Laurentia species and, as common characteristic, each manoenene derivative features terminal ethyl and
cis- or trans enyne units. In addition, all maneonene are bromochlorinated with chlorine at C5 and bromine at C13.
The biological activity of some of these compounds has been evaluated. Thus, compound 263 induces apoptosis and may be involved in regulation of programmed death in the initiation and propagation of inflammatory responses.160 Also this compound showed high potential as a natural insecticide against the beetle larvae Tribolium confusus and against the larvae of mosquito Culex pipiens.159 On the other hand, compounds 265 and 268 show antibacterial activity against some marine bacteria.161
Regarding the isomaneonenes, also isolated from Laurencia species, these compounds are all dibrominated at C-12 and C-13 showing, as the manoenene derivatives, the terminal ethyl and cis- or trans-enyne units (Figure 17).
To the best of our knowledge only the synthesis of cis-maneonenes A 258 and B 259 and trans- manoenene B 260 have been described in racemic form.
In both synthetic approaches (Scheme 33, a and b) the starting material was the readily available lactone 270.163 For the synthesis of 258 and 259 (Scheme 33a)164 lactone 270 was transformed into hemiacetal 271. Bromination of this masked carbonyl group afforded the bromoacetal ester 272 as a mixture of stereoisomers. This compound was converted into aldehyde 273 which reacts with 4-lithio-1-trimethylsilyl-butadiyne165 yielding a mixture of diastereoisomers 274 and 275. Partial catalytic hydrogenation166 of 274 gave 276 which, under pyrolytic conditions, gave a mixture of bromoenol ethers 277 and 278 in 20% and 30% yield respectively. After separation by column chromatography, independent reaction of 277 and 278 with (1-chloro-2-methyl-propenyl)-dimethylamine produced alcohols 279 and 280 which were desilylated to 258 and 259 respectively. For the synthesis of trans-maneonene B, the reduction of 275 was accomplished with LiAlH4 to give 281 which after the same reaction sequence furnished 260. (Scheme 33b).168
7. OTHER SESQUITERPENOIDS
Oxanorbornane derivatives 285-288 as well as the rearranged sesquiterpenoid 289 (Figure 18) have been isolated from different natural sources.169
On the other hand the eudesmanolide 290≠,170 has been isolated from Artemisia caerulescens and is probably generated by acid-catalyzed cyclization of shonachalin A 291 (Scheme 34).171
-----------------------------------
≠In reference 1, p. 13538 compound 290 was named as shonacholin B. However the correct structure of shonacholin B is 292 (stereochemistry not determined). See reference 170.
The oxanorbornane derivative 293 (1,4-epoxycadinane) was isolated from the brown alga Dilophus fasciola.172 The total synthesis of this compound (Scheme 35)173 constitutes a nice example of the application of the intramolecular furan Diels-Alder reaction (IMDAF) to the synthesis of epoxydecalin systems.174
The Friedel-Crafts alkylation of 2-methylfuran with (2E)-but-2-enal provided aldehyde 294 which was converted into iodide 295 in a three-steps sequence: reduction with NaBH4, conversion of the resulting alcohol into tosylate and reaction with NaI in acetone. Treatment of 295 with 2.2 equivalents of t-butyllithium followed by reaction with acroleyn provided allylic alcohol 296 as a mixture of diastereomers which were oxidized with DMSO, (COCl)2, Et3N (Swern) to furnished enone 297. Reaction of 297 with 1.1 equivalents of methylaluminium dichloride gave a mixture of cycloadducts 298 and 299 in ratio 298:299= 90:10.
After column chromatography, compound 298 was isolated in 88% yield. The hydrogenation of 298 followed by Wittig reaction of the resulting ketone 300 with the ylid of (methoxymethyl)triphenylphosphonium chloride afforded compound 301 as a mixture of E- and Z-diastereomers. This mixture was treated with dilute HCl in THF giving aldehyde 302. This compound was converted into methyl ketone 303 in two steps. Reaction of 303 with the ylid of methylentriphenyl phosphorane gave vinyl derivative 304 which was finally hydrogenated to 293.
Note that, in the major isomer 298, the methyl group at position 2 (axial) is syn oriented regarding the oxygen bridge (Figure 19).
It should be pointed out that, from ketone 300, a shorter-three steps route to 293 was tried. However, treatment of compound 305, generated by reaction of 300 with isopropyllithium in the presence of CuI, did not provide 293 under a variety of conditions (Scheme 36).
8. DITERPENOIDS
8.1 The dactylomelane group.
Diterpenes bearing the 3S,6S,7S,10R,11S-oxanorbornane 306 constitutes the so-called dactylomelane group.175 Up the date eight members of this group have been isolated from different natural sources (Table 2).
Regarding the biogenesis of the basic skeleton of dactylomelane it can be envisaged as resulting of a brominium ion-assisted C6-C11 cyclization of geranylgeraniol pyrophosphate 316 (via 317) to give intermediate 318. Further SN2-like reaction on bromine at C-10 give puctatene 308 which could diverges to the formation of dactylomlelol 307 or produce compounds 310-314 by the action of peroxidases (Scheme 37).175
Related with these compounds, metabolite 315 -dactylopyranoid (Figure 20)- has also been isolated from Aplysia dactylomella.178
A related pathway has been proposed for the biosynthesis of 315178 (Scheme 38). In this case the first bromine ion-assisted cyclization of 319 occurs between C10-C15 to give intermediate 320. On 320 takes place the formation of the pyrane ring of 315 through a second bromine ion-assisted cyclizations between the oxygen at C-3 and C-7 (321). Bromine ionization and 1,2-methyl shift form 322 in equilibrium (1,2-hydrogen-shift) with 323. Final intramolecular collapsing of 323 by cyclizations between oxygen at C-11 and C-14 generates the oxanorbornane subunit of 315.
Compound 307 has been proposed as a potentially useful defensive substance.176
8.2. Diterpenoids with ent-clerodane (neo-clerodane) and dolastane skeleton.
The basic structure of ent-clerodane (neo-clerodane) diterpenoids family, 324 (Figure 20) is named as decahydro-1,2-4a,5-tetramethyl-1-(3-methyl-pentyl)naphtalene.179 On the other hand the dolastane basic skeleton, 325 (Figure 21) is named as tetradecahydro-3a,5,8a-trimethyl-1-(1-methylethyl) benz[f]azulene.180
Teupestalin 326 (Figure 22) is a C-10 oxygenated neo-clerodane diterpenoid isolated from Teucrium pestalozzae.181 On the other hand the 1,4-epoxy-13-dolastene 327 has been isolated from a brown alga Dictyota sp.182 A second dolastane diterpene (1,4-epoxy-17-dolastene) 328 has been isolated from the brown alga Dilophus spiralis.183
9. TRITERPENOIDS
9.1. Cycloartane triterpenes.
Cycloartane triterpenoids184 feature the basic skeleton of 17-(1,5-Dimethyl-hexyl)-4,4,13,14-tetramethyl- tetradecahydro-cyclopropa[9,10]cyclopenta[a]phenanthrene 329 (Figure 23).
These compounds exist widely in nature185 and possess various bioactivities.186 Cimicifuga (currently Actaea in Europe and USA) species have been used for many years as medicinal herb worldwide. Previous chemical and biological studies on Cimicifuga species led to isolation of various bioactive compounds.187
In fact, the roots of some of these species such as C. foetida, C. dahurica, and C. heracleifolia are important elements of Traditional Chinese Medicine (TCM) and have been officially listed in the Chinese Pharmacopoeia. Several modified cycloartane triterpenoids isolated from these natural sources as well as semi- synthetic analogues showing a 7-oxanorbornane framework (230-244) are quoted in Tables 3-6 and Figure 24.
On the point of view of the biological activity of these compounds, it is known that active ingredients derived from traditional Chinese medicinal herbs have been reported to reverse multidrug resistance mediated by ATP-binding cassette sub-family B member 1 (ABCB1).ξ In this context, acerinol (234) was tested for its potential to modulate the ABCB1 transporter. The results indicate that this compound could be developing as a new multidrug resistance reversal agent.197
Compounds 230-233, 235 and its epimer at C-24 and 241 have been evaluated as potential antitumor promoters by inhibition of Epstein-Bar virusϒ early antigen concluding that these compounds might be valuable anti-tumor promoters.198
Finally, the antilipenic199 and antimalarial200 activities, among others201 have also been considered.
8.2. Baccharane triterpenes.
The baccharane-type triterpenes features the basic skeleton 245 (Figure 25)202 derived from octadecahydro chrysene.
----------------------------------------------------
ξThe ABCB1 is an important glycoprotein (170 kDa) of the cell membrane that pumps many foreign substances out of cells. In 2009, the first structure of a mammalian ABCB1 protein was solved. See: S. Aller, J. Yu, A. Ward, Y. Weng, S. Chittaboina, R. Zhug, P. M. Harrell, Y. T. Trinh, Q. Zhang, I. L. Urbatsch, and G. Chang, Science, 2009, 323, 1718.
ϒThe Epstein–Barr virus (EBV) is one of the most common viruses in human. It is associated with several particular forms of cancer. See: E. Maeda, M. Akahane, S. Kirgu, N. Kato, T. Yoshikawa, N. Hayashi, S. Aoki, M. Minami, H. Llozaki, M. Fukuyama, and K. Ohtomo, Jpn. J. Radiol., 2009, 27, 4. As consequence, many compounds that inhibit EBV-EA induction by tumor promoters have been shown to act as inhibitors of tumor promotion in vivo. See: J. Ishida, M. Kozuka, H. K. Wang, T. Konoshima, H. Tokuda, M. Okuda, X. Y. Mou, H. Nishino, N. Sakurai, K. H. Lee, and M. Nagai, Cancer Lett., 2000, 159, 135.
Baccharis oxide 246 (Figure 26) is a triterpene oxide first isolated203 from Baccharis halimifolia being also common in many Baccharis species204 This compound together campanulin (dendropanoxide, see below) are the only natural triterpenes showing a 3,10-oxide bridge in the A ring,205 within the family of the baccharane-type triterpenes.206
Biogenetically compound 246 arises from squalene oxide 247 via baccharenyl cation 248 which was in turn generated through a sequence involving intermediates 249 and 250. Successive 1,2-shifts and conformational changes afford cation 251. Final intramolecular attack of 3β-hydroxyl group yields 246. (Scheme 39).207
The ketone shinone 252208 appears to be derived from the same cation 251.207 Compound 252 was transformed into dehydrobaccharis oxide 253209 via formation of 3β,4β-epoxyshionane 254 followed by reaction with BF3·OEt2 at -30 ºC (Scheme 40).
In this reaction the following baccharane derivatives 255-258 (Figure 27) were also generated.
Recent studies have revealed the macrophag,211 trypanocidal,212 and antimicrobial213 activities of compound 246.
Dendropanoxide (campanulin) 259 (Figure 28) was first isolated from the leaves of Rhododendron grande214 as well as from others Rododendron species.215
On the biosynthetic point of view dandropanoxide arises from baccharenyl cation 248 via lupanyl cation 260. After a sequence of 1,2-shifts catalyzed by oxidosqualene cyclases (OSCs),≠,216 260 is transformed into 261. Quenching of 261with the hydroxyl group at C-3 finally produced 259 (Scheme 41).207
------------------------------------------------
≠The first committed step in triterpenoid biosynthesis is the cyclization of epoxysqualene into various triterpene alcohol isomers, a reaction catalyzed by oxidosqualene cyclases (OSCs). The different OSCs have characteristic product specificities, which are mainly due to differences in the numbers of high-energy intermediates the enzymes can stabilize. See reference 216.
A synthesis of 259 starting from friedelin 263 has been carried out217 as indicate in Scheme 42. Friedelin was transformed into friedel-3-ene 264 following previously reported synthetic protocol.218 Epoxidation of 264 with mCPBA gave the epoxides 265 and 266 in ratio 265:266 = 2:1. After separation, 266 was treated with BF3·OEt2 to give 259 in 22% yield.
In addition of 259, products 267-269 (Figure 29) were also identified in the last reaction depicted in Scheme 42.
Dendropanoxide 259 has been used as starting material for the preparation of other triterpenoids. Thus, acid treatment219 of 259 afforded a mixture of 270 and 271. After separation, 270220 was converted into daturadiol 272 or isomultiflorenol 273 (Scheme 43).221,222
The antiplasmodial223 and antidiabetic224 activity of 259 have been evaluated. On the other hand, this compound inhibits cell-proliferation and induces apoptosis in MG-63 human osteosarcoma cells.225 In this way, 259 constitutes a promising strategy for human osteosarcoma control.≠,226
9.3. The glycinoeclepins.
The glycinoeclepins A (274), B (275) and C (276) (Figure 30) are nortriterpenoids isolated from the soybean cyst nematode Heteropdena glycines (274)227 and from the dried root of the kidney bean Phasedus vulgaris (274-276).228 These compounds, especially 274, are potent hatching stimulus for the soybean cyst nematodes.ξ,229 The biosynthetic precursor of 274 appears to be the plant sterol cycloartenol 277230 and this was the basis for a biomimetic synthesis of 12-deoxyglycinoeclepin 278231 starting from abietospiran 279232 (Figure 30).
------------------------------------
≠Dendropanoxide induces autophagy through ERK1/2 activation in MG-63 human osteosarcoma cells and autophagy inhibition enhances dendropanoxide-induced apoptosis. See reference 226.
ξCyst nematodos (more than 40 species) are devastating pests for many crops. The infection causes various symptoms that may include chlorosis of the leaves and stems, root necrosis, loss in seed yield and suppression of root and shoot growth. By this reason the ecological eradication of the nematode constitutes a target of primary importance. See reference 229.
Until 1991 three total syntheses of 274 have been reported.233 These synthetic approaches were described in detail in several reviews234 and they will not be discussed here. A total synthesis of 274 based on the coupling of the fragments 280 and 281, Scheme 44, has been reported.235
The synthesis of fragment 281 (compound 296) was achieved as summarized in Scheme 45.
Optically pure γ-silyloxyenone 282 reacted with the anion generated from nitrile 283ϒ to give 285 with high diastereoselectivity. Transformation of 285 into ketone 290 was achieved using standard procedures. Compound 290 have the S-configuration at the C-12 position, opposite that 274. Attempts to invert alcohol 290 using Mitsunobu protocols failed and, by this reason, alcohol 290 was converted into ketone 291. Stereoselective reduction of 291 to alcohol 292 was carried out with excellent distereoselectivity (92:8, 88% yield) using LiEt3BH as bulky reducing agent. Compound 292 was finally converted into allyl tosylate 296 in seven steps and 73% overall yield.
Anionic specie 280 was generated from hydrazone 297 prepared in turn from diketone 298236 as depicted in Scheme 46.
-------------------------------------------
ϒNitrile 283 was prepared from glycerol monobenzyl ether 284 in four steps and 56% overall yield.
Final coupling of 296 and 297 afforded 303 which was transformed into 304 by Pd-catalyzed carbonylation reaction.237 Conversion of 304 into 274 was carried out in three steps and 77% yield from 304 (Scheme 47).
It should be pointed out that as consequence of the important biological activity of 274, different simpler analogues of this compound have been designed and synthesized. In the Figure 31 those which keep the 7-oxanorbornane moiety (307-310)238 were indicated together the benzenoid analogue 311.239,240
10. OTHERS TERPENOID DERIVATIVES
Subellinone 312 is a polyisoprenylated phloroglucinol derivative isolated from Garnicia subelliptica.241 This compound represents a novel type of phloroglucinol featuring a 10-oxatricyclo[3.3.1.13,9]decane skeleton. On the other hand compound 313, an inhibitor of farnesyl protein transferase known as SCH 58450 isolated from Streptomyces sp.,242 was initially formulated as 314. This initial proposed structure was later corrected. (Figure 32).243
11. CONCLUDING REMARKS
As discussed above, several organic molecules incorporating an 7-oxanorbornene skeleton not only have interesting molecular structures but also possess, in some cases, a variety of biological activities including cytotoxic, antimicrobial, enzyme-inhibitory, as well as potential ecological relevant functions, such as insecticidal activity. However, biological evaluations of these molecules have been limited. Therefore, much more extensive testing of these molecules seems warranted. Further investigation of the structure-activity relationships, as well as mode-of-action studies, could result in the discovery of promising analogues with potential clinical application.
Additional studies in this area could contribute to a better understanding of their mechanisms of action which would be beneficial in the ongoing development and structure optimization of this class of compounds, and could lead to the discovery of novel pharmacophores and heretofore news mechanisms of drug action. On the other hand, development of new strategies and efficient methods for the synthesis of these molecules are also necessary in light of the interesting structures and biological properties displayed by such compounds as well as, in some cases, the difficulties in obtaining large quantities of them from the natural sources.
12. ACKNOWLEDGEMENTS
The Spanish Ministerio de Ciencia e Innovación (MICINN) is thanked for financial support (project CTQ-2010-16170). S. R. is thankful to the Government of Spain for a Formación del Profesorado Universitario (FPU) Grant (AP20090051).
References
1. P. Vogel, J. Cossy, J. Plumet, and O. Arjona, Tetrahedron, 1999, 55, 13521. CrossRef
2. I. B. Masesane, Trends Org. Chem., 2010, 14, 13.
3. C. S. Schindler and E. M. Carreira, Chem. Soc. Rev., 2009, 38, 3222. CrossRef
4. For a review on the chemistry of the aeruginosins, see: K. Ersmark, J. R. Del Valle, and S. Hanessian, Angew. Chem. Int. Ed., 2008, 47, 1202. CrossRef
5. P. Vogel, Chimia, 2008, 62, 519. CrossRef
6. P. Vogel, Curr. Org. Chem., 2000, 4, 455. CrossRef
7. P. Vogel, Topics in Current Chem., 2008, 282 (Anthracycline Chemistry and Biology I), 187I.
8. I. Robina and P. Vogel, Synthesis, 2005, 675.
9. O. Arjona, A. G. Csákÿ, and J. Plumet, Eur. J. Org. Chem., 2003, 611; CrossRef N. Holub and S. Blechert, Chem. Asian J., 2007, 2, 1064. CrossRef
10. R. Gleiter, B. Esser, and S. C. Kornmayer, Acc. Chem. Res., 2009, 42, 1108. CrossRef
11. V. Lapinte, L. Fontaine, V. Montembault, I. Campistron , and D. V. Lapinte-Reyx, J. Mol. Catalysis, A, 2002, 190, 117.
12. Z. Zhang, O. Kelemen, M. A. van Santen, S. M. Yelton, A. Wendlandt, V. M. Sviripa, M. Bollen, L. Beullens, H. Urlaub, R. Luehrmann, D. S. Watt, and S. Stamm, J. Biol. Chem., 2011, 286, 10126.. CrossRef
13. J. P. Robiquet, Ann. Chem., 1810, 76, 302.
14. Selected, recent reviews: D. Perrotti and P. Neviani, Lancet Oncol., 2013, 14, 229; CrossRef P. Seshacharyulu, P. Pandey, K. Datta, and S. K. Batra, Cancer Lett., 2013, 335, 9; CrossRef R. Visconti, L. Palazzo, A. Pepe, R. Della Monica, and D. Grieco, Cell Cycle, 2013, 12, 17; CrossRef B. Ewald, J. Lesage, M. Goernemann, L. Beullens, M. Van Meervelt, and M. Bollen, FEBS J., 2013, 280, 584; CrossRef L. K. Nguyen, D. Matallanas, D. R. Croucher, A.von Kriegsheim, and B. N. Kholodenko, FEBS J., 2013, 280, 751; CrossRef V. Kolupaeva and V. Janssens, FEBS J., 2013, 280, 627; CrossRef W. Peti, A. Nairn, C. Angus, and R. Page, FEBS J., 2013, 280, 596; CrossRef P. Tsaytler and A. Bertolotti, FEBS J., 2013, 280, 766; CrossRef S. Munter, M. Kohn, and M. Bollen, ACS Chem. Biol., 2013, 8, 36; CrossRef T. Hunt, Adv. in Biol. Regulation, 2013, 53, 173. CrossRef
15. See, for instance: M. J. Graziano, A. L. Waterhouse, and J. E. Casida, Biochem. Biophys. Res. Commun., 1987, 149, 79; CrossRef W. Li, L. Xie, Z. Chen, Y. Zhu, Y. Sun, Y. Miao, Z. Xu, and X. Han, Cancer Sci., 2010, 101, 1226. CrossRef
16. C. E. Puerto Galvis, L. Y. Vargas Méndez, and V. V. Kouznetsov, Chem. Biol. Drug Design, 2013, 82, 477. CrossRef
17. M. Peng and Y. Yang, Acta Chromatogr., 2011, 23, 611; CrossRef M. A. Adatto, S. Halachmi, and M. Lapidoth, Current Prob. Dermat., 2011, 42, 97; H. Vidal Jr., C. J. Luiz Costa, L. Omar, and S. K. Tyring, J. Am. Acad. Dermat., 2012, 67, 331; J. O. Levitt, B. R. Keeley, and R. G. Phelps, J. Am. Acad. Dermat., 2013, 69, 254; CrossRef D. T. Pompei, K. S. Rezzadeh, K. V. Viola, D. H. Lee, D. O. Schairer, L. A. Chismar, and S. R. Cohen, J. Am. Acad. Dermat., 2013, 68, 1045. CrossRef
18. W. Sun, Z. Liu, and Y. Zhang, Int. J. Mol. Sci., 2013, 14, 1; CrossRef R. Maryam, R. A. Khan, Y. Zhang, and L. Ya, Int. J. Agric. Biol., 2013, 15, 993; M. Rashid, A. R. Khan, and Y. Zhang, J. Economic Entomology, 2013, 106, 2177; CrossRef B. E. Campbell, A. Hofmann, A. McCluskey, and R. B. Gasser, Biotechnology Adv., 2011, 29, 28. CrossRef
19. A. Rudo, H. U. Siehl, K. P. Zeller, S. Berger, and D. Sicker, Chem. in Unserer Zeit, 2013, 47, 310. CrossRef
20. For selected, recent reviews, see: L. Deng, J. Dong, and W. Wang, Mini-Rev. Med. Chem., 2013, 13, 1166; CrossRef L. P. Deng, J. Dong, H. Cai, and W. Wang, Curr. Med. Chem., 2013, 20, 159; CrossRef L. Deng and S. Tang, Expert Opinion on Therapeutic Patents, 2011, 21, 1743. CrossRef
21. Y. C. Chen, S. C. Chang, M. H. Wu, K. A. Chuang, J. Y. Wu, W. J. Tsai, and Y. C. Kuo, Life Sci., 2009, 84, 218. CrossRef
22. A. Enz, G. Zenke, and E. Pombo-Villar, Bioorg. Med. Chem. Lett., 1997, 7, 2513. CrossRef
23. For a review on endothall, see: R. C. Lord, Med. Biochem., 2001, 1, 315; For others selected references concerning this compound, see also: J. Bajsa, Z. Pan, D. Zhiqiang, E. Franck, D. K. Owens, and S. O. Duke, Pest. Biochem. Physiol., 2012, 102, 38; CrossRef S. Tresch and J. Schmotz, Pest. Biochem. Physiol., 2011, 99, 86. CrossRef
24. Z. Liu, J. Zhang, J. Yuan, Y. Dang, C. Yang, X. Chen, J. Xu, and L. Yu, Mol. Biol. Rep., 2005, 32, 41. CrossRef
25. Y. Y. Wang, R. D. Hu, Q. Y. Lin, Y. L. Zhao, and N. Wang, Asian J. Chem., 2010, 22, 5993.
26. M. R. Reithofer, S. M. Valiahdi, M. Galanski, M. A. Jakupec, V. B. Arion, and B. K. Keppler, Chemistry Biodivers., 2008, 5, 2160. CrossRef
27. F. L. Yin, J. J. Zou, L. Xu, X. Wang, and R. C. Li, J. Rare Earths, 2005, 23, 596.
28. S. K. Li, Q. Y. Lin, L. Tx, Y. J. Wang, and D. Chen, Chinese J. Struct. Chem., 2010, 29, 1632.
29. F. Zhang, W. Z. Zhu, Q. Y. Lin, W. D. Guo, L. L. Zhang, and S. K. Li, J. Rare Earths, 2011, 29, 297. CrossRef
30. N. Wang, Q. Y. Lin, J. Feng, Y. L. Zhao, Y. J. Wang, and S. K. Li, Inorg. Chim. Acta, 2010, 363, 3399. CrossRef
31. N. Wang, Q. Y. Lin, Y. H. Wen, L. C. Kong, S. K. Li, and F. Zhang, Inorg. Chim. Acta, 2012, 384, 345. CrossRef
32. Q. Y. Li, Y. Y. Wang, Y. L. Zhao, D. M. Yan, F. J. Wang, and F. Zhang, J. Coord. Chem., 2011, 64, 920. CrossRef
33. F. Zhang, X. L. Zhang, Q. Y. Lin, X. L. Zheng, L. L. Zhang, Q. Y. Yang, and J. Y. Gu, J. Fluoresc., 2012, 22, 1395. CrossRef
34. F. Zhang, X. L. Zheng, Q. Y. Lin, P. P. Wang, and W. J. Song, Inorg. Chim. Acta, 2013, 394, 85. CrossRef
35. F. Zhang, Q. Y. Lin, S. K. Li, Y. L. Zhao, P. P. Wang, and M. M. Chen, Spectrochim. Acta, 2012, 98A, 436. CrossRef
36. F. Zhang, Q. Y. Lin, W. L. Hu, W. J. Song, S. T. Shen, and P. Gui, Spectrochim. Acta, 2013, 110A, 100. CrossRef
37. Isolation : J. S. Chandra and M. Sabir, Indian J. Pharm. Sci., 1978, 40, 97; Structural determination: R. J. Bochis and M. H. Fisher, Tetrahedron Lett., 1968, 16, 1971; CrossRef Absolute configuration: M. G. Meter, G. Snatzke, F. Snatzke, K. N. Nagarajan, and H. Schmid, Helv. Chim. Acta, 1974, 57, 32. CrossRef
38. R. Raj, R. Kaleysa, and P. A. Kurup, Indian J. Med. Res., 1968, 56, 1818.
39. T. Nakatani, T. Konishi, K. Miyahara, and N. Noda, Chem. Pharm. Bull., 2004, 52, 807. CrossRef
40. T. Nakatani, K. Jimpo, and N. Noda, Chem. Pharm. Bull., 2007, 55, 92. CrossRef
41. P. K. Guha, R. Poi, and A. Bhattaacharyya, Phytochemistry, 1990, 29, 2017. CrossRef
42. Z. Wang, H. L. Leng, K. Sha, and J. Liu, lit. cit. in 1a, reference 46 quoted in this paper.
43. A. McCluskey, S. P. Ackland, E. Gardiner, C. C. Walkom, and J. A. Sakoff, Anti-Cancer Drug Design, 2001, 16, 291.
44. For selected references see: A. McCluskey, C. C. Walcom, M. C. Bowyer, S. P. Ackland, E. Gardiner, and J. A. Sakoff, Bioorg. Med. Chem. Lett., 2001, 11, 2941; CrossRef S. H. L. Kok, C. H. Chui, W. S. Lam, J. Chen, F. Y. Lau, S. M. Raymond, G. Y. M. Cheng, W. K. Tang, Y. T. N. Teo, F. Cheung, C. H. Cheng, A. S. C. Chang, and J. C. O. Tang, Int. J. Mol. Med., 2006, 18, 1217; S. H. Kok, C. H. Chui, W. S. Lam, J. Chen, F. Y. Lau, A. S. M. Wong, G. Y. M. Cheng, P. B. S. Lai, T. W. T. Leung, M. W. Y. Michael, J. C. O. Tang, and A. S. C. Chen, Bioorg. Med. Chem. Lett., 2007, 17, 1155. CrossRef
45. I. J. Tseng, S. Y. Sheu, P. Y. Lin, J. A. Lee, K. L. Ou, and L. W. Lee, J. Exp. Clin. Med., 2012, 4, 280. CrossRef
46. I. J. Tseng, S. Y. Sheu, Y. T. Chen, C. Y. Huang, C. T. Lin, and P. Y. Lin, Chem. Pharm. Bull., 2012, 60, 1453. CrossRef
47. H. Guenther, E. Ramstad, and H. G. Floss, J. Pharm. Sci., 1969, 58, 1274; CrossRef W. D. Woggon, S. A. Hauffe, and H. Schmid, J. Chem. Soc., Chem. Comm., 1983, 272; J. P. McCormick, J. E. Carrel, and J. P. Doom, J. Am. Chem. Soc., 1986, 108, 8071; CrossRef J. P. McCormick and J. E. Carrel, Environ. Sci. Res., 1992, 44 (Secondary-Metabolite Biosynthesis and Metabolism), 261.
48. For a first, unsuccessful attempt, see: F. von Bruchhausen and W. Bersch, Arch. Pharm. Ber. Deusch. Pharm. Ges., 1928, 266, 697.
49. G. Stork, E. E. van Tamelen, L. I. Friedman, and A. W. Burgstahler, J. Am. Chem. Soc., 1953, 75, 384. CrossRef
50. G. Schenck and R. Wirtz, Naturwissenshaften, 1953, 40, 531. CrossRef
51. W. G. Dauben, C. R. Kessel, and K. H. Takemura, J. Am. Chem. Soc., 1980, 102, 6893 and references therein. CrossRef
52. P. A. Grieco, J. J. Nunes, and M. S. Gaul, J. Am. Chem. Soc., 1990, 112, 4595. CrossRef
53. C. E. Puerto Galvis, L. Y. Vargas Mendez, and V. V. Kouznetsov, Chem. Biol. Drug Des., 2013, 82, 477. CrossRef
54. A. McCluskey, M. A. Keane, C. C. Walcom, M. C. Bowyer, A. T. R. Sim, D. J. Young, and J. A. Sakoff, Bioorg. Med. Chem. Lett., 2002, 12, 391. CrossRef
55. H. Kotsuki, H. Nishizawa, S. Kitagawa, O. Masamitsu, H. Yamasaki, K. Matsuoka, and T. Tokoroyama, Bull. Chem. Soc. Jpn., 1979, 52, 496. CrossRef
56. Y. Baba, N. Hirukawa, and M. Sodeoka, Bioorg. Med. Chem., 2005, 13, 5164. CrossRef
57. D. B. Rydberg and J. Meinwald, Tetrahedron Lett., 1996, 37, 1129. CrossRef
58. W. G. Dauben, J. Y. L. Lam, and Z. R. Guo, J. Org. Chem., 1996, 61, 4816. CrossRef
59. Selected, recent reviews : A. A. Bekhit, A. Hymete, A. E. A. Bekhit, A. Damtew, and H. Y. Aboul-Enein, Mini-Rev. Med. Chem., 2010, 10, 1014; CrossRef A. Schmidt and A. Dreger, Curr. Org. Chem., 2011, 15, 1423; CrossRef G. G. Kumar, K. Vikas, and K. Vinod, Res. J. Chem. Environ., 2011, 15, 90; F. K. Keter and J. Darkwa, BioMetals, 2012, 25, 9; CrossRef V. Kumar, K. Kaur, G. K. Gupta, G. Girish, and A. K. Sharma, Eur. J. Med. Chem., 2013, 69, 735. CrossRef
60. K. B. Umesha, R. K. M. Lokanatha, and K. K. Ajay, Indian J. Chem., 2002, 41B, 1450.
61. O. Wallach, Justus Liebigs Ann. Chem., 1907, 356, 197. CrossRef
62. "Encyclopedia of Food and Color Additives“, ed. by G. A. Burdock, Vol. 1, CRC Press, 1997, p. 590.
63. General references: D. E. Metzler and C. Metzler “Biochemistry: The Chemical Reactions of Living Cells”, Vol. 2, Academic Press, 2003, Chapter 22, p. 1232; J. Gershenzam and R. B. Croteau, “Lipids Metabolism in Plants”, ed. by T. S. Moore Jr., CRC Press, 1993, pp. 339-388; J. Bohlmann, C. L. Steele, and R. Croteau, J. Biol. Chem., 1997, 272, 21784; CrossRef J. Bohlmann, G. Meyer-Gauen, and R. Croteau, Proc. Natl. Acad. Sci. USA, 1998, 95, 4126; CrossRef M. L. Wise, T. J. Sauvage, E. Katahira, and R. Croteau, J. Biol. Chem., 1998, 273, 14891; CrossRef D. C. Williams, D. J. McGarvey, E. Katahira, and R. Croteau, Biochemistry, 1998, 37, 12213. CrossRef
64. J. Degenhardt, T. G. Köllner, and J. Gershenzonb, Phytochemistry, 2009, 70, 1621. CrossRef
65. E. J. L. Lana, K. A. Rocha da Silva, I. V. Kozhevnikov, and E. V. Gusevskaya, J. Mol. Catalysis, 2006, 259A, 99. CrossRef
66. See reference 64, p. 1050.
67. J. A. Klocke, M. V. Darlington, and M. F. Balandrin, J. Chem. Ecol., 1987, 13, 2131. CrossRef
68. V. Sfara, E. N. Zerba, and R. A. Alzogaray, J. Med. Entomol., 2009, 46, 511. CrossRef
69. F. P. Schiestl and D. W. Roubik, J. Chem. Ecol., 2004, 29, 253. CrossRef
70. U. R. Juergens, U. Dethlefsen, G. Steinkamp, A. Gillissen, R. Repges, and H. Vetter, Resp. Med., 2003, 97, 250. CrossRef
71. J. Lackie, A Dictionary of Biomedicine, Oxford University Press, 2010.
72. U. R. Juergens, M. Stöber, and H. Vetter, Eur. J. Med. Res., 1998, 3, 508; U. Juergens, T. Engelen, K. Racké, M. Stöber, A. Gillissen, and H. Vetter, Pulmonary Pharmacol. Therapeut., 2004, 17, 281. CrossRef
73. W. Kehrl, U. Sonnemann, and U. Dethlefsen, Laryngosc., 2004, 114, 738. CrossRef
74. F. A. Santos and V. S. Rao, Phytotherapy Res., 2000, 14, 240. CrossRef
75. H. Moteki, H. Hibasami, Y. Yamada, H. Katsuzaki, K. Imai, and T. Komiya, Oncology Reports, 2002, 9, 757.
76. W. Jäger, “Metabolism of Terpenoids in Animal Models and Humans”, Handbook of Essential Oils: Science, Technology and Applications, ed. by K. Husnucan Baser and G. Buchbauer, CRC Pres, 2009, pp. 214-215. CrossRef
77. For selected reviews see: S. O. Duke, J. G. Romagni, and A. M. Rimando, Weed Res., 2000, 40, 99; CrossRef S. O. Duke and A. Oliva, Allelopathy, 2004, 201; G. S. Buttar and N. Aggarwal, Pestology, 2005, 29, 27; A. F. M. Barton, B. Dell, and A. R. Knight, J. Agric. Food Chem., 2010, 58, 10147. CrossRef
78. S. N. Grag, L. N. Misra, and S. K. Aggarwal, Phytochemistry, 1989, 28, 634. CrossRef
79. J. P. N. Rosazza, J. J. Steffens, F. S. Sariaslani, A. Goswani, J. M. Beale Jr., S. Reeg, and R. Chapman, Appl. Environ. Microbiol., 1987, 53, 2482.
80. J. P. N. Rosazza, A. Goswani, W. G. Liu, F. S. Sariaslani, J. J. Steffens, R. P. Steffek, J. M. Beale, Jr., R. Chapman, and S. Reeg, Develop. Industry Microbiol. Series, 1988, 29, 181.
81. M. Miyazawa, Y. Nana, K. Yamamoto, and H. Kameoka, Chem. Express, 1991, 6, 771.
82. H. Hamada, K. Ishihara, N. Nakajima, H. Hamada, H. J. Williams, and A. I. Scott, Lett. Org. Chem., 2004, 1, 171. CrossRef
83. W. G. Liu, A. Goswami, R. P. Steffek, R. L. Chapman, F. S. Sariaslani, J. J. Steffens, and J. P. N. Rosazza, J. Org. Chem., 1988, 53, 5700. CrossRef
84. K. Grossmann, J. Hutzler, S. Tresch, N. Christiansen, R. Looser, and T. Ehrhardt, Pest Manag. Sci., 2012, 68, 482. CrossRef
85. A. Lopukhima, M. Dettenberg, E. W. Weiler, and H. Hollander-Czytko, Plant Physiol., 2001, 126, 1678; CrossRef E. J. Lee and P. J. Facchini, Plant. Physiol., 2011, 157, 1067; CrossRef D. Riewe, M. Koochi, J. Lisec, M. Pfeiffer, R. Lippmann, J. Scheimeichel, L. Willmitzer, and T. Altmann, Plant J., 2012, 71, 850 and references cited in these papers. CrossRef
86. P. Reddy and S. Urban, Phytochemistry, 2009, 70, 250. CrossRef
87. A. Hausmann and G. Sandmann, Fungal Genet. Biol., 2000, 30, 147; CrossRef A. F. Estrada, D. Maier, D. Scherzinger, J. Avalos, and S. Al-Babili, Fungal Genet. Biol., 2008, 45, 1497. CrossRef
88. G. Romagni, G. Joanne, S. O. Duke, O. Stephen, and F. E. Dayan, Plant Physiol., 2000, 123, 725. CrossRef
89. For a review on the biological function of asparagine synthetase in plants, see: L. Gaufichon, M. Reisdorf-Cren, S. J. Rothstein, F. Chardon, and A. Suzuki, Plant Sci., 2010, 179, 141. CrossRef
90. P. B. Gomes, M. L. Feitosa, M. I. G. Silva, E. C. Noronha, B. A. Moura, E. T. Venâncio, E. R. V. Rios, D. Pergentino de Sousa, S. M. Mendes de Vasconcelos, M. M. F. Fonteles, and F. C. Florenco de Sousa, Pharmacol. Biochem. Behav., 2010, 96, 287. CrossRef
91. S. G. Griffin, S. G. Wyllie, J. L. Markham, and D. N. Leach, Flavour and Fragrance J., 1999, 14, 322. CrossRef
92. A. Ahad. M. Aqil, K. Kohli, Y. Sultana, M. Mujeeb, and A. Ali, Curr. Drug Deliver., 2011, 8, 213. CrossRef
93. K. Endo and H. H. Kino, Can. J. Chem., 1984, 62, 2011. CrossRef
94. E. Mousouri, E. Milieu, and P. Magiatis, J. Agr. Food Chem., 2014, 62, 660. CrossRef
95. P. K. Shashikumar and P. Vijayendra, J. Essent. Oil Res., 1993, 5, 659. CrossRef
96. L. Caglioti, H. Naef, D. Arigoni, and O. Jeger, Helv. Chim. Acta, 1958, 41, 2278; CrossRef See also: L. Caglioti, H. Naef, D. Arigoni, and O. Jeger, Helv. Chim Acta, 1959, 42, 2557; CrossRef M. I. Nassar, E. A. Abu-Mustafa, and A. A. Ahmed, Pharmazie, 1995, 50, 766; A. Ghosh, A. Banerji, S. Mandal, and J. Banerji, Nat. Prod. Comm., 2009, 4, 1023; M. R. Cha, Y. H. Choi, C. W. Choi, Y. S. Kim, S. H. Ryu, Y. H. Kim, and U. S. Choi, Planta Med., 2011, 77, 52. CrossRef
97. M. Iranshahi, P. Arfa, M. Ramezani, M. R. Jaafari, H. Sadeghian, C. Bassarello, S. Piacente, and C. Pizza, Phytochemistry, 2007, 68, 554. CrossRef
98. L. Teng, G. Z. Ma, L. Li, L. Y. Ma, and X. Q. Su, Chem. Nat. Compd., 2013, 49, 606. CrossRef
99. J. M. Rollinger, T. M. Steindl, D. Schuster, I. Kirchmair, K. Amain, E. P. Elmerer, T. Langer, H. Stupper, P. Wutzler, and M. Schmidtke, J. Med. Chem., 2008, 51, 842; CrossRef C. L. Lee, L. C. Chiang, L. H. Cheng, C. C. Liaw, M. H. Abd El-Razek, F. R. Chang, and Y. C. Wu, J. Nat. Prod., 2009, 72, 1568. CrossRef
100. J. H. Lee, S. Choi, Y. Lee, H. J. Lee, K. H. Kim, K. S. Alin, H. Bae, H. J. Lee, E. O. Lee, K. S. Ahn, S. H. Ryu, J. Lue, and H. S. Kim, Mol. Cancer Therapeut., 2010, 9, 388.
101. E. E. van Tamelen, Acc. Chem. Res., 1975, 8, 152; CrossRef A. Eschenmoser and D. Arigoni, Helv. Chim. Acta, 2005, 3011; CrossRef R. A. Yoder and J. N. Johnston, Chem Rev., 2005, 105, 4730. CrossRef
102. E. E. van Tamelen and R. M. Coates, Chem. Comm., 1966, 413; E. E. van Tamelen and R. M. Coates, Bioorg. Chem., 1982, 11, 171. CrossRef
103. E. A. Abu-Mustafa, F. K. El-Bay, and M. B. Fayez, J. Pharm. Sci., 1971, 60, 788; CrossRef See also: G. Cravotto, G. Balliano, B. Robaldo, S. Boso-Oliaro, S. Chimichi, and M. Boccalini, Bioorg. Med. Chem. Lett., 2004, 14, 1931. CrossRef
104. R. P. Hanzlik, Org. Synth., 1977, 56, 112. CrossRef
105. C. J. Rhodes, Ann. Rep. Prog. Chem., 2007, 103C, 287; CrossRef E. F. Sousa-Aguiar, F. Eduardo, F. E. Triguero, and F. M. Z. Zotin, Catal. Today, 2013, 115; CrossRef and 218.
106. C. Tsangarakis, E. Arkoudis, C. Raptis, and M. Stratakis, Org. Lett., 2007, 9, 583; CrossRef C. Tsangarakis, C. Raptis, E. Arkoudis, and M. Stratakis, Adv. Synt. Catal., 2008, 350, 1587. CrossRef
107. F. W. J. Demmitz, C. Philippini, and R. A. Raphael, J. Org. Chem., 1995, 60, 5114. CrossRef
108. T. Mukaiyama and N. Iwasawa, Chem. Lett., 1981, 10, 29. CrossRef
109. O. Hofer and H. Gereger, Liebigs Ann. Chem., 1985, 1136. CrossRef
110. F. O. Bohlmann, C. Zdero, and H. Kaptein, Liebigs Ann. Chem., 1968, 17, 186. CrossRef
111. M. Aziz and F. Rouessac, Tetrahedron, 1988, 44, 101; CrossRef X. Zhang, A. Archelas, A. Meou, and R. Furstoss, Tetrahedron: Asymmetry, 1991, 2, 247. CrossRef
112. M. Leblanc, G. Ferey, F. Rouessac, and M. Aziz, Acta Cryst., 1988, C44, 1262.
113. R. M. Coates and L. S. Melvin, Jr., Tetrahedron, 1970, 26, 5699. CrossRef
114. For a short, comprehensive review see : S. Genovese and F. Epifano, Curr. Drugs Targets, 2011, 12, 381. CrossRef
115. E. E. van Tamelen and T. J. Curphey, Tetrahedron Lett., 1962, 121. CrossRef
116. See reference 113 and: M. Aziz and F. Rouessac, Tetrahedron Lett., 1987, 28, 2579. CrossRef
117. See reference 113 and: A. Mueller, W. R. Abraham, and K. Kieslich, Bull. Soc. Chim. Belg., 1994, 103, 405. CrossRef
118. A. T. Sneden, Synlett, 1993, 313. CrossRef
119. Z. H. Gao, B. Liu, and W. D. Z. Li, Tetrahedron, 2005, 61, 10734. CrossRef
120. M. P. DiFazio and A. T. Sneden, J. Nat. Prod., 1990, 53, 1357. CrossRef
121. R. Kaiser and D. Lamparsky, Helv. Chim. Acta, 1978, 61, 373. CrossRef
122. J. Casadebaig, Y. Pelissier, C. Marion, M. Milhau, and J. M. Bessiere, J. Essent. Oils, 2000, 677; A. Boulanger and J. Crouzet, Food Chem., 2001, 74, 209. CrossRef
123. Y. B. Bose, A. Altintas, O. Tugay, T. Uysal, B. Demirci, K. Ertugrul, and C. H. K. Baser, Asian J. Chem., 2010, 22, 7159.
124. J. A. Pino and R. Marbot, J. Agric. Food Chem., 2001, 49, 5880. CrossRef
125. M. LeRoux, J. C. Cronge, B. V. Burger, and J. Elizabeth, J. Agric. Food Chem., 2012, 60, 2657. CrossRef
126. M. P. DiFazio, W. W. Wallace, and A. T. Sneden, Heterocycles, 1989, 29, 2391. CrossRef
127. A. B. K. Rao, P. S. N. Murthy, M. K. Chakrabonty, and T. Fujimori, Tobacco Res., 1987, 13, 14.
128. Y. Tagaki, T. Fujimori, T. Hata, K. Tuyoshi, H. Kaneko, and K. Kato, Agric. Biol. Chem., 1980, 44, 705. CrossRef
129. X. W. Li, Z. T. Guo, Y. Zhao, Z. Zhao, and J. F. Hu, Phytochemistry, 2010, 71, 682. CrossRef
130. J. Shitamoto, K. Matsunami, H. Otsuka, T. Shinzato, and Y. Takeda, Chem. Pharm. Bull., 2010, 58, 1026. CrossRef
131. T. Kato, H. Kondo, Y. Kitano, G. Hata, and Y. Takagi, Chem. Lett., 1980, 757. CrossRef
132. J. A. Findlay and W. D. MacKay, Can. J. Chem., 1971, 49, 2369; CrossRef W. Cocker, K. J. Crowley, and K. Srinivason, J. Chem. Soc., Perkin Trans. 1, 1973, 2485. CrossRef
133. I. Brito, M. Cueto, A. Díaz-Marrero, J. Darias, and A. San Martín, J. Nat. Prod., 2002, 65, 946. CrossRef
134. A. G. González, J. D. Martin, M. Norte, R. Pérez, P. Rivera, J. Z. Ruano, M. L. Rodríguez, J. Fayos, and A. Perales, Tetrahedron Lett., 1983, 24, 4143. CrossRef
135. M. Suzuki, M. Daitoh, C. S. Variappan, T. Abe, and M. Masuda, J. Nat. Prod., 2001, 64, 597. CrossRef
136. E. Breitmaier “Terpenes: Flavors, Fragances, Pharmaca, Pheromones”, Wiley, 2006 pp. 24-26 and 45; CrossRef “Total Synthesis of Natural Products: A Sesquidecade of Sesquiterpenes: Total Synthesis, 1980-1994“. Part B: Bicyclic and Tricyclic Sesquiterpenes, ed. by D. Goldsmith, M. C. Pirrung, A. T. Morehead, and B. G. Young, Wiley, 2007, Volume 11.
137. K. A. Mohammed, C. F. Hossain, L. Zhang, R. K. Bruick, Y. D. Zhou, and D. G. Nagle, J. Nat. Prod., 2004, 67, 2002. CrossRef
138. See for instance: M. Quintero, N. Mackenzie, and P. A. Brennan, Eur. J. Surg. Oncol., 2004, 30, 465 and references therein. CrossRef
139. M. E. Jung and G. Y. Jamie, Tetrahedron Lett., 2008, 49, 4962; CrossRef M. E. Jung and G. Y. Jamie, J. Org. Chem., 2009, 74, 8739. CrossRef
140. K. F. Podraza and R. L. Basfield, J. Org. Chem., 1989, 54, 5919. CrossRef
141. Compound 179 was first prepared in 1929. See: O. Diels and K. Alder, Ber. Deutsch. Chem. Ges., 1929, 62B, 554. CrossRef
142. B. Bonnlander, B. Baderschneider, M. Messerer, and P. Winterhalter, J. Agric. Food Chem., 1998, 46, 1474. CrossRef
143. S. Mukherjee, A. P. Scopton, and E. J. Corey, Org. Lett., 2010, 12, 1836. CrossRef
144. M. K. Brown and E. J. Corey, Org. Lett., 2010, 12, 172 and references therein. CrossRef
145. E. N. Pitsinos, N. Athinaios, and V. P. Vidali, Org. Lett., 2012, 14, 4666. CrossRef
146. D. Liu, E. Canales, and E. J. Corey, J. Am. Chem. Soc., 2007, 129, 1498. CrossRef
147. H. Guth, Helv. Chim. Acta, 1996, 79, 1559. CrossRef
148. A. G. Chittiboyina, G. M. Kumar, P. B. Carvalho, Y. Liu, Y. D. Zhao, D. G. Nogle, and M. A. Avery, J. Med. Chem., 2007, 50, 6299. CrossRef
149. D. S. Caine and R. F. Collson, Synlett, 1995, 503; CrossRef D. S. Caine and M. A. Paige, Synlett, 1999, 1391. CrossRef
150. M. N. Paddon-Row, N. G. Rondan, and K. N. Houk, J. Am. Chem. Soc., 1982, 104, 7162. CrossRef
151. A. G. Chittiboyina, P. Peddikotla, M. A. Avery, and I. A. Khan, J. Org. Chem., 2013, 78, 9223. CrossRef
152. H. Buschmann and H. D. Scharf, Synthesis, 1988, 827. CrossRef
153. R. H. Crabtree and M. W. Davis, J. Org. Chem., 1986, 51, 2655; CrossRef K. H. Hopmann and A. Bayer, Organometallics, 2011, 30, 2483. CrossRef
154. R. H. Crabtree, Acc. Chem. Res., 1979, 12, 331; CrossRef J. M. Brown, Angew. Chem., Int. Ed. Engl., 1987, 26, 190; CrossRef T. L. Church and P. G. Anderson, Coord. Chem. Rev., 2008, 252, 513; CrossRef See also. H. U. Blaser, “Applications of Iridium Catalysts in the Fine Chemical Industry. Iridium Complexes in Organic Synthesis”, ed. by L. A. Oro and C. Claver, Wiley, 2009. Chapter 1, pp. 1-14; See in particular pp. 8-12.
155. A. Murai “Biosynthesis of Cyclic Bromoethers from Red Algae. Comprehensive Natural Products Chemistry”, ed. by D. Barton, K. Nakanishi, and O. Meth-Cohn, Elsevier, 1999. Vol. 1, p. 303; CrossRef V. M. Dembiitsky, A. G. Tolstikov, and G. A. Tolstikov, Chem. Sustainable Develop., 2003, 11, 329.
156. S. M. Waraszkiewicz, H. H. Sun, and K. L. Erickson, Tetrahedron Lett., 1976, 3021. CrossRef
157. S. M. Waraszkiewicz, H. H. Sun, K. L. Erickson, J. Fiver, and J. Clardy, J. Org. Chem., 1978, 43, 3194. CrossRef
158. H. H. Sun, S. M. Waraszkiewicz, and K. L. Erickson, Tetrahedron Lett., 1976, 4227. CrossRef
159. Z. S. Abou-Elnaga, W. M. Alarif, and S. S. Al-Lihaibi, Clean. Soil, Air, Water, 2011, 39, 787. CrossRef
160. S. E. N. Ayyad, K. O. Al-Footy, W. M. Alarif, T. R. Sobahi, S. A. Bassaif, M. S. Makki, A. M. Asiri, A. Y. Al Halwani, A. F. Badria, and F. A. A. Badria, Chem. Pharm. Bull., 2011, 59, 1294. CrossRef
161. C. S. Variappan, M. Daitoh, M. Suzuki, T. Abe, and M. Masuda, Phytochemistry, 2001, 58, 291. CrossRef
162. C. S. Variappan, M. Suzuki, T. Abe, and M. Masuda, Phytochemistry, 2001, 58, 507.
163. C. L. D. Jennings-White, A. B. Colmes, and P. R. Raithby, J. Chem. Soc., Chem. Comm., 1979, 542.
164. A. B. Colmes, C. L. D. Jennings-White, and D. A. Kendrick, J. Chem. Soc., Chem. Comm., 1983, 415.
165. E. N. Garbisch, J. Org. Chem., 1965, 30, 2109. CrossRef
166. A. B. Colmes, R. A. Raphael, and K. N. Wellard, Tetrahedron Lett., 1976, 1539.
167. B. Haveaux, A. Dekoker, M. Rens, A. R. Sidote, J. Toye, and L. Ghosez, Org. Synth., 1980, 59, 26.
168. B. Haveaux, A. Dekoker, M. Rens, A. R. Sidote, J. Toye, and L. Ghosez, Org. Synth., 1980, 59, 26.
169. Compound 285 from Vetiver oils: S. P. Weyerstahl, H. Marschall, U. Splittgerber, and D. Wolf, Liebigs Ann., 1996, 1195; P. Weyerstahl, H. Marschall, U. Splittgerber, D. Wolf, and H. Surburg, Flavour Fragrance J., 2000, 15, 395; CrossRef P. Campagnat, G. Figueredo, J. C. Chalchat, A. P. Carnat, and J. M. Bessiere, J. Essent., Oil. Res., 2006, 18, 416; CrossRef Compound 286 from Ambrosia artemisides : J. Jakupovic, M. Jaensch, F. Bohlmann, and M. O. Dillon, Phytochemistry, 1988, 27, 3551; CrossRef Compound 287 from Varoi nijareiensis : E. Cabrera, A. García-Granados, and M. A, Quecuty, Phytochemistry, 1988, 27, 183; CrossRef Compound 288 from Artemisia barrelieri : J. A. Marco, J. F. Sanz, A. Yuste, M. Carda, and J. Jakupovic, Phytochemistry, 1991, 30, 3661. CrossRef
170. J. F. Sanz and J. A. Marco, Phytochemistry, 1990, 29, 2913. CrossRef
171. J. A. Marco, J. F. Sanz-Cervera, V. García-Lliso, L. R. Domingo, M. Carda, S. Rodríguez, F. López-Ortiz, and J. Lex, Liebigs Ann., 1995, 1837. CrossRef
172. E. Fattorusso, S. Magno, and L. Mayol, Gazz. Chim. Ital., 1979, 109, 589.
173. C. Rogers and B. A. Keay, Tetrahedron Lett., 1989, 30, 1349; CrossRef C. Rogers and B. A. Keay, Can. J. Chem.,1993, 71, 611. CrossRef
174. Review: B. A. Keay and I. R. Hunt, “Aspects of the intramolecular Diels-Alder reaction of furan dienes leading to the formation of epoxydecalin systems”, Advances in Cyloaddition, 1999, 6, 173, ed. by M. Harmata. Elsevier.
175. J. J. Fernández, M. L. Souto, L. V. Gil, and M. Norte, Tetrahedron, 2005, 61, 8910. CrossRef
176. D. M. Estrada, J. L. Ravelo, C. Ruiz-Pérez, J. D. Martín, and X. Solans, Tetrahedron Lett., 1989, 30, 6219. CrossRef
177. J. A. Findlay and G. Li, Can. J. Chem., 2002, 80, 1697. CrossRef
178. M. Wessels, G. M. Koenig, and A. D. Wright, J. Nat. Prod., 2002, 80, 1697.
179. Review: T. Tokoroyama, Synthesis, 2000, 611. CrossRef
180. Reviews: M. Hiesermann and H. Hemboldt, “Natural products Synthesis: Targets, Methods, Concepts. Topics in Curr. Chem., 2005, 243, 73, ed. by J. Mulzer, J. D. Connolly, and R. A. Hill, “Dictionary of Terpenoids”, Springer, 991, Vol. 2: Di- and Higher Terpenoids, p. 655.
181. M. C. De la Torre, B. Rodríguez, M. Bruno, G. Savona, F. Piozzi, A. Perales, M. R. Torres, and O. Servettaz, Phytochemistry, 1990, 29, 2229. CrossRef
182. A. Gallardo, E. Manta, J. D. Martín, C. Pérez, R. Pérez, and M. L. Rodríguez, Rev. Latinoamericana de Química, 1988, 19, 86.
183. E. Ioannou, C. Vagias, and V. Roussis, Marine Drugs, 2013, 11, 1104. CrossRef
184. Reviews: M. I. Isaev, M. B. Gorovits, and N. K. Abubavikov, Chem. Nat. Prod., 1985, 21, 399; I. Saleem, “Cycloartane Triterpenoids”, VDM Verlag, 2010; S. S. Azimova, “Natural Compounds: Cycloartane Triterpenoids and Glycosides”, Springer, 2013.
185. J. A. Compton, A. Culham, and S. L. Jury, Taxon, 1998, 47, 593; CrossRef J. A. Compton, A. Culham, J. G. Gibbings, and S. L. Jury, Biochem. Syst. Ecol., 1998, 26, 185. CrossRef
186. Z. E. Tian, Y. E. Sun, P. G. Xiao, and E. X. Wu, Recent Prog. Med. Plants, 2011, 31, 49; H. I. C. Lowe, N. J. Toyang, C. T. Watson, and J. Bryant, Br. J. Med. Med. Res., 2014, 4, 1802; CrossRef R. Tundis, F. Menichini, and M. R. Loizzo, Recent Insights into the Emerging Role of Triterpenoids in Cancer Therapy. Antitumor Activity of Triterpenoids: The Cycloartene Group. Studies in Natural Products Chemistry, ed. by Atta-ur-Rahman, Elsevier, 2014, Vol. 41, Chap. 1, pp. 2-6.
187. J. X. Lim and Z. Y. Zu, Curr. Med. Chem., 2006, 13, 2927. CrossRef
188. Pharmacopoeia of Chinese People’s Republic; Chemical Industry: Beijing, 2005; Vol. 1, pp. 68-69.
189. First isolation: “Constituents of Cimifuga species. III. Constituents of Cimifuga acerina. 3. Structure of Acerinol”. Abstract from Scifinder. T. Takemoto, G. Kusano, and N. Yamamoto, Yakugaku Zasshi, 1967, 87, 1489; “Studies on the constituents of Cimifuga spp. XII. A revised struture of acerinol and the structures of the related compounds”. Abstract from Scifinder. G. Kusano, A. Uchida, Y. Murakami, N. Sakurai, and T. Takemoto, Yakugaku Zasshi, 1976, 96, 321; Y. Nian, H. Zhu, W. R. Tang, Y. Luo, J. Du, and M. H. Qiu, J. Nat. Prod., 2013, 76, 896. CrossRef
190. Y. R. Liu, Z. J. Wu, C. T. Li, F. M. Xi, L. N. Sun, and W. S. Chen, Planta Med., 2013, 79, 301 and references therein. CrossRef
191. J. X. Li, S. Kadota, M. Hattori, S. Yosimachi, M. Shiro, N. Oogami, H. Mzuno, and T. Namba, Chem. Pharm. Bull., 1993, 41, 832. CrossRef
192. Crystal structure: R. Y. Liu, C. T. Li, S. Qin, L. N. Sun, and Z. J. Wu, Z. Kristallogr.-New Cryst. Struct., 2011, 226, 169.
193. G. Kusano, S. Hojo, Y. Kondo, and T. Takemoto, Chem. Pharm. Bull., 1977, 25, 3182. CrossRef
194. A Kusano, M. Takahira, M. Shibano, T. Miyase, T. Okuyama, and G. Kusano, Heterocycles, 1998, 48, 1003. CrossRef
195. G. Kusano, S. Nozoe, Z. Taira, and T. Takemoto, Heterocycles, 1983, 20, 1951. CrossRef
196. H. Hemmi, F. Kitame, N. Ishida, G. Kusano, Y. Kondo, and S. Nozoe, J. Pharm. Dyn., 1979, 2, 339. CrossRef
197. D. L. Liu, Y. J. Li, N. Yao, J. Xu, Z. S. Chen, A. Yiu, C. X. Zhang, W. C. Ye, and D. M. Zhang, Eur. J. Pharmacol., 2014, 733, 34. CrossRef
198. N. Sakurai, M. Kozuka, H. Tokuda, Y. Nobakuni, J. Takayasu, H. Nishino, A. Kusano, G. Kusano, M. Wagei, Y. Sakurai, and K. H. Lee, Bioorg. Med. Chem., 2003, 11, 1137. CrossRef
199. A. Kusano, M. Shibano, G. Kusano, and T. Miyase, Chem. Pharm. Bull., 1996, 44, 2078. CrossRef
200. M. Takahara, A. Kusano, M. Shibano, G. Kusano, K. Koizumi, R. Suzuki, H. S. Kim, and Y. Wataya, Biol. Pharm. Bull., 1998, 21, 823. CrossRef
201. A. Yawata, Y. Matsuhashi, H. Kato, K. Uemura, G. Kusano, J. Ito, T. Chikuma, and H. Hojo, Eur. J. Pharm. Sci., 2009, 38, 355. CrossRef
202. E. Britmaier, “Terpenes: Importance, General Structure and Biosynthesis”. Wiley, 2006, p. 96. CrossRef
203. I. Anthonsen, T. Bruun, E. Hemmer, D. Holme, A. Lamvik, E. Sund, and N. A. Sorensen, Acta Chem. Scand., 1970, 24, 2479; CrossRef Crystal structure: F. Mo, Acta Crystallogr., 1973, 29B, 796.
204. F. Bohlmann and C. Zdero, Chem. Ber., 1976, 109, 1450; CrossRef F. Bohlmann, W. Kanupf, R. M. King, and H. Robinson, Phytochemistry, 1979, 18, 1011; CrossRef F. Bohlmann, C. Zdero, R. M. King, and H. Robinson, Phytochemistry, 1979, 18, 1533; CrossRef F. Bohlmann, C. Zdero, H. Robinson, and R. M. King, Phytochemistry, 1979, 18, 1993; CrossRef F. Bohlmann, C. Zdero, M. Grena, A. K. Dhar, H. Robinson, and R. M. King, Phytochemistry, 1981, 20, 281; CrossRef F. Bohlmann, N. Kramp, M. Grenz, H. Robinson, and R. M. King, Phytochemistry, 1981, 20, 1907; CrossRef F. Bohlmann, S. Banerjee, J. Jakupovic, M. Grenz, L. N. Misra, G. Schemeda-Hirschmann, R. M. King, and H. Robinson, Phytochemistry, 1985, 24, 511; CrossRef J. Jakupovic, R. N. Baruali, F. Bohlmann, R. M. King, and H. Robinson, Tetrahedron, 1985, 41, 4537; CrossRef U. Warning, F. Bohlmann, V. H. Sánchez, S. E. Del Rio, and X. A. Domínguez, Rev. Latinoamericana de Química, 1986, 51, 155; A. P. Rivera, F. Faini, and M. Castillo, J. Nat. Prod., 1988, 51, 155; CrossRef C. Zdero, F. Bohlmann, J. C. Solomon, R. M. King, and H. Robinson, Phytochemistry, 1989, 28, 531. CrossRef
205. Review: R. Xu and G. C. Fazio, Phytochemistry, 2004, 65, 261.
206. See T. Kushiro and Y. Ebizuka, “Triterpenes. Comprehensive Natural Products. II. Chemistry and Biology, ed. by C. A. Townsed and Y. Ebizuka, Elsevier, 2010, Vol. 1, Ch., 18, p. 685.
207. M. Shibuya, A. Sagara, A. Sayito, T. Kushiro, and Y. Ebizuka, Org. Lett., 2008, 10, 5071. CrossRef
208. T. Takahashi, T. Moriyama, Y. Tanahashi, and G. Ourisson, Tetrahedron Lett., 1967, 31, 2991. CrossRef
209. K. Tachibana, M. Tori, Y. Moriyama, T. Tsuji, and T. Takahashi, Bull. Chem. Soc. Jpn., 1977, 50, 1552. CrossRef
210. S. Yamada, S. Yamada, K. Tachibana, Y. Moriyama, Y. Tanahashi, T. Ysuyuki, and T. Takahashi, Bull. Chem. Soc. Jpn., 1976, 49, 1134 and references therein.
211. F. Missima, A. A. da Silva, G. A. Nunes, P. C. P. Bueno, J. P. B. de Sousa, J. K. Bastos, and J. M. Sforcin, J. Pharm. Pharmacol., 2007, 59, 463. CrossRef
212. A. A. da Silva, P. C. P. Bueno, L. E. Gregório, M. L. A. da Silva, S. Alburquerque, and J. K. Bastos, J. Pharm. Pharmacol., 2004, 56, 1195. CrossRef
213. M. Herrera-Martínez, M. V. Ramírez-Mares, E. Burgueño-Tapia, E. Cedillo-Portugal, C. Miron-Enriquez, and B. Hernández-Carlos, Rev. Latinoamericana de Química, 2012, 40, 165.
214. S. Rangaswami and P. Venkateswarku, Proc. Indian Acad. Sci., 1965, 62A, 224; Crystal structure: J. D. White, J. Fayos, and J. Clardy, J. Chem. Soc., Chem. Comm., 1973, 357; F. Mo, Acta Crystallogr., 1977, 33B, 641; CrossRef F. Mo, Acta Crystallogr., 1982, 38B, 2166. CrossRef
215. P. Sengupta and A. K. Dey, J. Indian Chem. Soc., 1970, 47, 713; N. C. Gupta, Curr. Sci., 1978, 47, 768; I. M. Chung, M. Y. Kim, S. D. Park, W. H. Park, and H. I. Moon, Phytother. Res., 2009, 23, 1634. CrossRef
216. K. U. Wendt, Angew. Chem. Int. Ed., 2005, 44, 3966. CrossRef
217. M. Tori , K. Tachibana, S. Yamada, T. Tsuyoki, and T. Takahashi, Bull. Chem. Soc. Jpn., 1977, 50, 469. CrossRef
218. E. J. Corey and J. J. Ursprung, J. Am. Chem. Soc., 1956, 78, 504; CrossRef G. Brownley, F. S. Spring, R. Stevenson, and W. S. Strachan, J. Chem. Soc., 1956, 2419. CrossRef
219. M. Tori, M. Takai, Y. Matsumoto, Y. Moriyama, T. Tsuyuki, T. Takahashi, H. Ohnishi, A. Itai, and Y. Iitaka, Bull. Chem. Soc. Jpn., 1984, 57, 2490 and references therein. CrossRef
220. Isolated from Datura innoxia. See: M. Kocór, J. S. Pyrek, C. K. Atal, K. L. Bedi, and B. R. Sharma, J. Org. Chem., 1973, 38, 3685. CrossRef
221. M. Takai, M. Tori, T. Tsuyuki, T. Takahashi, A. Itai, and Y. Iitaka, Chem. Pharm. Bull., 1984, 32, 2464. CrossRef
222. Isolated from different species such as Cucumis, Bryonia, Zanthoxylum and Pelargonium. See T. Itoh, T. Shigimoto, N. Shimizu, T. Tamura, and T. Matsumoto, Phytochemistry, 1982, 21, 2414 and references therein. CrossRef
223. I. M. Chung, M. Y. Kim, S. D. Park, W. H. Park, and H. I. Moon, Phytother. Res., 2009, 23, 1634. CrossRef
224. H. I. Moon, Hum. Exp. Toxicol., 2011, 30, 870. CrossRef
225. In vitro cultivation of human tumor tissues: A. Billiau, J. J. Cassiman, D. Willems, M. Verhelst, and H. Heremans, Oncology, 1975, 31, 257. CrossRef
226. J. W. Lee, K. S. Kim, H. K. An, C. H. Kim, H. I. Moon, and Y. C. Lee, PLoS One, 2013, 8, e83611/1. Note: PLOS ONE (originally PLoS ONE) is an open access peer-reviewed scientific journal published by the Public Library of Science since 2006.
227. T. Masamune, M. Anetai, A. Furuzawa, M. Takasugi, H. Matsue, K. Kobayashi, S. Ueno, and N. Katsui, Bull. Chem. Soc. Jpn., 1987, 60, 981; CrossRef T. Masamune, A. Furuzawa, A. Furusaki, M. Ikura, H. Matsue, T. Kaneko, A. Abiko, N. Sakamoto, N. Tanimoto, and A. Murai, Bull. Chem. Soc. Jpn., 1987, 60, 1001; CrossRef Review: T. Masamune, Nat. Prod. Biol. Act., Naito Found. Symp., 1986, pp. 25-32, ed. by H. Imora.
228. A. Furuzawa, H. Matsue, M. Ikura, and T. Masamune, Tetrahedron Lett., 1985, 26, 5539. CrossRef
229. Review: H. Grisebach, Comments on Agric. Food Chem., 1987, 1, 27.
230. T. Masamune, M. Anetai, M. Takasugi, and N. Katsui, Nature, 1982, 297, 495; CrossRef A. Furuzawa, A. Furusaki, M. Ikura, and T. Masamune, J. Chem. Soc., Chem. Comm., 1985, 222.
231. E. J. Corey and B. C. Hong, J. Am. Chem. Soc., 1994, 116, 3149; CrossRef This synthetic sequence was described and discussed in reference 1, p. 13544.
232. W. Steglich, M. Klaar, L. Zechlin, and H. J. Hecht, Angew. Chem., Int. Ed. Engl., 1979, 18, 698. CrossRef
233. A. Murai, N. Tanimoto, N. Sakamoto, and T. Masamune, J. Am. Chem. Soc., 1988, 110, 1985; CrossRef H. Watanabe and K. Mori, J. Chem. Soc., Perkin Trans. 1, 1991, 2919; CrossRef E. J. Corey and I. N. Houpis, J. Am. Chem. Soc., 1990, 112, 8997. CrossRef
234. See reference 1, pp. 13540-13544 and: K. Mori and H. Watanabe, Pure Appl. Chem., 1989, 61, 543; CrossRef A. Murai, Pure Appl. Chem., 1989, 61, 393; CrossRef K. Mori, Chem. Script., 1989, 24, 395.
235. Y. Shiina, Y. Tomata, M. Miyashita, and K. Tanino, Chem. Lett., 2010, 39, 835. CrossRef
236. Y. Lu, G. Barth, K. Kieslich, P. D. Strong, W. L. Duax, and C. Djerassi, J. Org. Chem., 1983, 48, 4549. CrossRef
237. A. Murai, N. Takimot, N. Sakamoto, and T. Masamune, J. Am. Chem. Soc., 1988, 110, 1985. CrossRef
238. A. Murai, M. Ohkita, T. Honma, N. Tanimoto, S. Araki, and A. Fukuzawa, Chem. Lett., 1992, 2103. CrossRef
239. S. Giroux and E. J. Corey, Org. Lett., 2008, 10, 5617. CrossRef
240. G. A. Kraus, L. Yander, S. Leuw, G. Tylk, and D. H. Soh, J. Agric. Food Chem., 1996, 44, 1548; CrossRef G. A. Kraus and P. K. Choudury, Eur. J. Org. Chem., 2004, 2193 and references therein; CrossRef See also: S. A. Snyder, Nature Chem., 2011, 3, 422. CrossRef
241. Y. Fukuyama, A. Kaneshi, N. Tani, and M. Kodama, Phytochemistry, 1993, 33, 483. CrossRef
242. D. Phife, R. W. Patton, R. L. Berrie, R. Yaborough, M. S. Puar, M. Patel, W. R. Bishop, and S. J. Coval, Tetrahedron Lett.,1995, 36, 6995. CrossRef
243. D. Phife, R. W. Patton, R. L. Berrie, R. Yaborough, M. S. Puar, M. Patel, W. R. Bishop, and S. J. Coval, Tetrahedron Lett., 1996, 37, 5527; CrossRef D. Wiege, Aust. J. Chem., 1996, 49, 669.