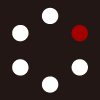
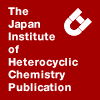
HETEROCYCLES
An International Journal for Reviews and Communications in Heterocyclic ChemistryWeb Edition ISSN: 1881-0942
Published online by The Japan Institute of Heterocyclic Chemistry
e-Journal
Full Text HTML
Received, 30th June, 2014, Accepted, 24th September, 2014, Published online, 3rd October, 2014.
DOI: 10.3987/COM-14-S(K)73
■ Structural and Ecotoxicological Profile of N-Alkoxymorpholinium-Based Ionic Liquids
Robert Salchner, Gerhard Laus, Simone Haslinger, Volker Kahlenberg, Klaus Wurst, Doris E. Braun, Stefan Vergeiner, Holger Kopacka, Herwig Schottenberger,* Alan Puckowski, Marta Markiewicz, Stefan Stolte, and Sven Nerdinger*
Faculty of Chemistry and Pharmacy, Leopold-Franzens University, Innrain 80, 6020 Innsbruck, Austria
Abstract
Alkylation of N-methylmorpholine N-oxide (1, NMMO) using dialkyl sulfates gave N-alkyloxymorpholinium alkyl sulfates 2 and 3 (alkyl = Me, Et), which were subjected to ion metathesis. The tetrachloroferrates(III) 4 and 5 were found to be advantageous precursors for the chlorides 6 and 7 which were converted to six other salts. In summary, 13 new quaternary salts were prepared (IO4 8 and 9, BF4 10, N3 11, AcO 12, (MeO)2PO2 13, PF6 14, Tf2N 15) and one hydrate 7·H2O. Eleven X-ray crystal structures were determined. Nine salts had melting points below 100 °C, thus qualifying as ionic liquids (ILs), and three more below 110 °C. In addition, catalytic hydrogenation gave two protic ionic liquids (PILs), N-methylmorpholinium alkyl sulfates 16 and 17 (alkyl = Me, Et). This reductive degradation was also performed in aqueous solution. Ecotoxicological examinations of 3, 4, and 5 showed minor potential to interact with acetylcholinesterase (AChE), moderate acute toxicity towards rat leukemia IPC-81 cells, no adverse effect towards green algae Scenedesmus vacuolatus, but no ready biodegradability in sewage sludge.INTRODUCTION
Ionic liquid-related materials science is proliferating, and its impact on potential applications can be attributed to the tuneability of the organic cations. The virtually unlimited structural diversity of ILs is reflected by their wide spectrum of physicochemical properties available for industrial processes.1,2 In particular, ILs can be used (a) as designer solvents, (b) in separation technology, (c) in electrochemistry. However, a set of key prerequisites has to be met for the successful advancement of ILs towards practical applicability. They should be (a) inexpensive, (b) accessible by a simple synthesis, (c) non-(eco)toxic,3 biodegradable,4,5 non-bioaccumulating,6 (d) recyclable, (e) patent-free.
These requirements are met by the derivatives of N-methylmorpholine N-oxide (NMMO) described below. Only a few examples of quaternary salts derived from this industrial cellulose solvent have been reported.7 A patent-free situation can even promote the launch of known ILs into new industrial processes, thus leading to new patent disclosures.8 In this context, it is worth noting, that the number of ILs containing N-alkoxy substituents is almost negligible and N-O-embodiments are rarely claimed in new inventions.9 To our knowledge, such claims have not been mentioned in patents dealing with ILs containing weakly coordinating, hydrophobic anions.10,11 Empirical concepts for the design of small molecules for biodegradability12 originate from research in the detergent and pesticide industry, but they are also applicable for ILs.13 The following molecular features generally result in poor biodegradation and should be avoided: halohydrocarbons, extensive chain branching, tertiary amine, nitro, nitroso, azo, arylamino groups, and polycyclic aromatic hydrocarbons.12 Biocompatibility should not interfere with the functional groups required for the respective technical tasks. For example, the influence of ester or ether functions was found to be beneficial in terms of acute toxicity,6 and the incorporation of oxygen into ILs proved to be essential for optimized sequestration of sour greenhouse and flue gases such as CO213 and SO2.14 Any heteroatoms incorporated in side chains are lowering hydrophobicity, a crucial point, as some applications of ILs deal with the extraction of pollutants from wastewater. Such processes require ILs that are sufficiently hydrophobic to form a biphasic system with water. As a conflicting result, for environmentally more acceptable ILs a much higher potential of dissemination in aqueous effluents has to be anticipated.15
In the present investigation we report the optimized synthesis and characterization of 4-methoxy- and 4-ethoxy-4-methylmorpholinium salts, supplemented by hydrogenolytic chemical degradation to protic ILs (PILs), a sub-group with outstanding environmental safety.16 In addition, we include a preliminary (eco)toxicity and biodegradability evaluation of their tetrachloroferrates, a popular17 anion of moderate hydrophilicity, already utilized for the recovery of ILs from wastewater.18
RESULTS AND DISCUSSION
Synthetic considerations
Based on preliminary results,7 we herein extend the preparative chemistry of new ILs derived from NMMO (Scheme 1). With regard to the known hazards of pure NMMO compared to the harmless7 NMMO hydrate (1), dialkyl sulfates represent the alkylation reagents of choice, since they are compatible with aqueous reaction conditions due to their preference of amine and N-oxide alkylation in favor of R–OH alkylation (R3N > R3N+–Oˉ >>> R–OH / H2O). Thus, the N-alkyloxy-N-methylmorpholinium alkyl sulfates 2 and 3 were obtained in quantitative yield. The isolation of quaternary ammonium salts from aqueous reaction mixtures by precipitation / extraction with weakly coordinating anions from aqueous solutions is a well established preparative tool. In view of the fact that the replacement of expensive hexafluorophosphates with hydrochloric acid / ferric chloride, or tetrachloroferrates, respectively, has already proved applicable for quaternary alkoxyimidazolium cations,11 we were curious whether this low hydrophilicity of FeCl4ˉ could also be exploited for the work-up of N-alkyloxy-N-methylmorpholinium ions from aqueous dialkyl sulfate-based alkylation mixtures.
Fortunately, the resulting morpholinium tetrachloroferrates 4 and 5 also form binary phase systems with water. Conversion to the chlorides 6 and 7 by aqueous ammonia was straightforward. Interestingly, the N-methoxy derivative 6 was obtained as anhydrate, whereas the N-ethoxy derivative 7 was reproducibly obtained as monohydrate. These chlorides are ideal substrates for ion metathesis. Thus, from the appropriate sodium or ammonium salts a series of new ILs was prepared, i. e. periodates 8 and 9, tetrafluoroborate 10, azide 11, acetate 12, and dimethyl phosphate 13. Their insolubility in water allows direct precipitation of the hexafluorophosphate 14 as a solid and the bis(trifluoromethanesulfonyl)imide (‘triflimide’) 15 as a liquid. In the latter case, addition of hydrochloric acid was necessary to prevent carry-over of ethyl sulfate into the organic phase.11
The excellent chemical and thermal stability of many ILs unfortunately implies their contingent persistence in the environment, necessitating methods of their cleanup after usage prior to disposal. So far, the focus of chemical degradation studies in aqueous media was laid on oxidative and thermal degradation of ILs.4 In the case of morpholine, the intended circumvention of degradative inertness of the heterocyclic cation core by appending alkyl side chains via weak N–O single bond linkages as a predetermined scission site offers the opportunity of reductive disintegration. This concept could be successfully demonstrated by a standard hydrogenation step proceeding with exceptional ease and selectivity (Scheme 2). Hence, upon fragmentation of such ILs by hydrogenolysis they are convertible to parent structures of NMMO, for which a dedicated biodegradation study has been published.19
Crystal structures
Remarkably, crystallographic methods of characterization, especially single crystal structure determinations, are powerful tools for the evaluation of the typical contributions of interactions in ILs. Series of related salts of a specific cation (or anion) have proven to be of particular value for this purpose. By systematically comparing different crystal structures that are obtained by varying the respective counter-ion, it is possible to elucidate the influence of coulombic forces, of steric and charge delocalization effects, and of heteroatoms with lone pairs as additional hydrogen bond acceptor moieties on the packing architectures under investigation.20 Such categorization concepts have already been applied in studies of N-methylmorpholinium salts.21 Consequently, we were tempted to proceed likewise for the closely related N-alkoxy-N-alkylmorpholinium salts, since the study of cation-anion interactions is conclusively providing insight into features controlling solid-liquid phase interconversions as well.
Eleven X-ray crystal structures were determined. Phase purity was assessed by powder X-ray diffraction (PXRD) of the bulk material and confirmed by Pawley fits (see Supporting Information, Figures S1–S10 and Tables S1–S10). In all cases, the morpholinium ring adopts a regular chair conformation, and the alkyloxy groups occupy axial positions. There are numerous interionic contacts shorter than the sum of van der Waals radii (see Supporting Information, Table S11). Thus, in the crystal structures of the tetrachloroferrates(III) 4 and 5, C–H···Cl interactions are dominant (Figures 1 and 2).
In the structures of the anhydrous chlorides 6 and 7, each cation forms a network of weak C–H···Cl– hydrogen bonds to surrounding anions. In addition, several short C–H···O contacts are observed (Figures 3 and 4). In the rather complex structure of the chloride monohydrate 7·H2O, which contains two independent ion pairs, not only C–H···Cl– and C–H···O contacts, but also O–H···Cl– hydrogen bonds are found (Figure 5). In the structures of the periodates 8 and 9 (Figures 6 and 7), each cation donates hydrogen bonds to six surrounding anions. Short C–H···N contacts are observed between the cations and the terminal nitrogen atoms of the anions in the structure of the azide 11 (Figure 8).
The choice of fluorine-containing anions such as tetrafluoroborate, hexafluorophosphate, and triflimide provided an opportunity to study non-covalent hydrogen-fluorine interactions. After some controversy, it has been concluded that short C–H···F contacts between oppositely charged molecules are genuine interionic hydrogen bonds.22
In the structure of salt 10, two independent cations are linked by bridging tetrafluoroborate anions (Figure 9). In crystals of the hexafluorophosphate 14 (Figure 10), each cation forms C–H···F contacts with seven anions. The weakly coordinating triflimide ion in salt 15 adopts the anti conformation (Figure 11) with a C–S···S–C torsion angle of 169.5°. Remarkably, the triflimide anion exhibits not only the expected C–H···O interactions but also a C–H···N contact.
General aspects of biosafety and environmental benignity
Dating back to the 1940's, archetypical, medium to long chain substituted imidazolium and dihydroimidazolium compounds, including numerous room temperature ILs (RTILs),23 were shown to have surface tension depressing and correlative bacteriostatic properties, hereby providing synergistic value for combating various microbial invasions. The applicability of these salts as topical antiseptics prompted the originators to file respective patent applications.24 This obvious leitmotif of structure–toxicity relationship, namely the side chain length effect,25 also termed detergent effect,6 has been found to be consistent in all levels of biological complexity.
In particular, an increase in alkyl-chain length, or lipophilicity, was observed to be interrelated with an increase in the rate of degradation, as well as an increase in toxicity, which indicates a conflict of aims between minimizing the toxicity and maximizing the biodegradability. At any rate, the introduction of functional polar groups to the alkyl chain has been shown to generally reduce the toxicity of ILs and increase the biodegradation efficiency to some extent. Such findings, with respect to conscious and sustainable design, indicate the possibility of tailoring ILs by coupling suitable functional groups to their structure, which in turn might lead to compounds of higher intrinsic safety.3,4
Regardless of any possible measures of biocontainment, the complex matter of eco(cyto)activity is adding up to indispensable parameters for task-specific optimization.26 Hence, only those IL candidates which fulfill balanced requirements of technical suitability and environmental benignity should be selected as working fluids for commercial applications.
According to the published literature, the structure of the cation of ILs is alleged to be the most important factor affecting their toxicity.27 However, to allow reason to prevail, such “statistical” toxicity assessments of ILs have, with the exception of fluorous organic anions,28 not been reported explicitly for ion combinations consisting of anions being “infamous” for their extremely high toxicity, e. g. azides, cyanides, or their related complex metallates.29 When turning to extremes, an ultimate, yet illustrative exaggeration could be the hypothetical conversion of a notorious chemical celeb, the warfare agent VX, into (non-volatile) ILs in analogy to conceptually well elaborated synthetic methods.30 It seems rather expectable to obtain salts containing the S-(2-N,N-diisopropylaminoethyl) methylphosphonothioate anion, (CAS-RN [73207-98-4]), known as EA 2192, a hydrolysis by-product of VX which exhibits similar toxicity to VX itself.31 To give particular evidence for common or alternate (including reductive) pathways of biodegradation of ILs, a test regimen with activated sludge (see experimental section) is usually the method of choice since the strains present in such a microbial consortium are well acquainted with xenobiotic compounds32 and versed to cope with unprecedented metabolic challenges. However, for exotic anthropogenic chemical structures such as some extremely toxic and persistent phosphonothioates, only a specific singular system like glycerophosphodiesterase from Enterobacter aerogenes may be sufficient for effective enzymatic decomposition at all.33 Recently, some ILs have even been recognized to induce new fungal metabolite biosynthetic pathways.34
In general conclusion, the partial biodegradability of ILs by alteration or removal of a side chain functionality35 should only be considered a “green accomplishment” in exceptional cases, since leaving the quaternized cation core unaltered does not justify the assessment of overall reduced toxicity. This holds true especially for aromatic heterocyclic systems such as imidazolium-based ILs.6 Furthermore, the presence of ester functions renders the ILs more readily (but only partially) biodegradable, especially for long alkyl side-chains in the cation, but, on the other hand, leads to enzymatic degradation with the formation of reaction products that may accumulate.4
The constitution of the head group of ILs clearly demonstrated higher toxicity/cytotoxicity of the aromatic cations, relative to quaternary ammonium and alicyclic ions (including morpholinium).36
In comparison with pyrrolidinium- and piperidinium-based ILs, the morpholinium family turned out to be the least toxic due to the incorporation of oxygen in the ring.3 The significant effect of the triflimide anion was again observed, since substituting the halide in N-butyl-N-methylmorpholinium halides by triflimide increased, up to one hundred times, its toxicity against V. fischeri and S. vacuolatus.36
Biodegradability and ecotoxicological considerations regarding NMMO derivatives
As rationalized above, the “benign by design” approach and the idea of green or sustainable chemistry are perfectly applicable to ILs. Again, the structural variability of ILs permits systematic investigations and a conscious3 and sustainable4,37 structural design leading (potentially) to ILs with reduced hazard to humans and the environment. With respect to the latter, especially N-methylmorpholinium-based ILs substituted with short N-alkyloxy substituents appear promising since their known N-alkyl-N-methylmorpholinium analogs (with quaternizing substituents ≤ butyl) showed low acute toxicities towards e. g. IPC-81 rat cells,38 bacteria Vibrio fischeri,36 green algae Pseudokirchneriella subcapitata39 and Scenedesmus vacuolatus,36 water flea Daphnia magna39 and zebrafish Danio rerio39 when tested with halide as counterions. In terms of biodegradability morpholinium compounds were found to show less recalcitrance towards aerobic biodegradation processes than imidazolium compounds. However, a complete degradation was only found for N-methylmorpholinium compounds with hydroxyl-containing side chains.40 For NMMO, biodegradation takes place in several steps involving reduction to N-methylmorpholine followed by demethylation to morpholine and final ring cleavage.19
For the tentative evaluation of the environmental benignity of 4-methoxy- and 4-ethoxy4-methylmorpholinium ILs, test systems of different complexity were chosen for a first estimate of the toxicity of selected salts. The results, expressed as half maximal inhibitory concentration (IC50) or half maximal effective concentration (EC50), are displayed in Table 1. Additional literature data on monocationic relatives are shown for comparison. We chose 1-butyl-1-methylmorpholinium bromide (CAS-RN [75174-77-5]) and 1-methyl-3-octylimidazolium bromide (CAS-RN [61545-99-1]) as reference compounds for lower and higher acute IL toxicity.
Enzyme inhibition
The enzyme inhibition test with acetylcholinesterase (AchE) is an important biological marker in (eco)toxicology for evaluating the influence of chemicals on the central nervous system of organisms. For both test compounds IC50s are in a similar range as values found for 1-butyl-1-methylmorpholinium bromide indicating a generally low inhibition potential to this enzyme. From a structure-activity point of view, a low inhibition potential was expected since previous studies demonstrated that a certain lipophilicity is the key feature defining the inhibitory potential of IL cations.41 The type of anion generally does not affect the inhibition potential of ILs.41 Due to their moderate hydrophobicity, the investigated morpholinium compounds exhibit a minor potential to interact with the enzyme.
Cell toxicity with IPC-81 and Scenedesmus vacuolatus
As a second step, cellular test systems with rat leukemia and green algae were employed. In-vitro testing with the rat leukemia cell line IPC-81 was used to screen for effects on basal cell functions and structures of cells. The other cellular test in this study was performed with the limnic green algae Scenedesmus vacuolatus. Algae are primary producers and have a high relevance in the aquatic food chain. They are thus important test organisms for the hazard assessment of chemicals and for environmental legislation. Both tests have been proven useful for determining the acute toxicological hazards of ILs.42–44 The alkoxymorpholinium compounds exhibited a moderate cytotoxicity towards IPC-81 cells with EC50s that are one order of magnitude higher than the values found for the toxic reference 1-methyl- 3-octylimidazolium bromide, but also one order of magnitude lower than 1-butyl-1-methylmorpholinium bromide. From previous studies a clear trend of greater cytotoxicity with increasing hydrophobicity was found,38,45 therefore it would rather be expected that the alkoxymorpholinium compounds would have lower toxicity than 1-butyl-1-methylmorpholinium bromide. However, a deviation from this behavior was previously observed for e. g. ethoxymethyl substituted ILs where the toxicity towards IPC-81 cells was higher than expected from their hydrophobicity.38 Moreover, the higher than expected toxicity might be partially explained by the intrinsic effect of FeCl4ˉ that can be demonstrated when comparing EC50 values of 5 (≈ 1500 µM) and 3 (3243 µM). Ethyl sulfate is considered non-toxic in this test system.43 None of the alkoxymorpholinium compounds showed any statistically significant adverse effects towards Scenedesmus vacuolatus in test concentrations up to 1000 µM, and the observed effective concentrations are more than six orders of magnitude higher than the values found for 1-methyl-3-octylimidazolium bromide. For ILs containing FeCl4ˉ a clearly improved algae growth (in comparison with the control without test chemical) was observed (data not shown). Apparently FeCl4ˉ serves as additional Fe3+–source that is an essential trace element within the media.
Primary biodegradation tests
Primary biodegradation experiments were conducted in order to screen the biodegradability of alkoxymorpholinium compounds and imidazole as the reference compound. The cation concentration was monitored by ion chromatography (IC) during a test period of 28 days. The primary degradation of imidazole was complete within 7 days indicating the general biological activity of the inoculums (data not shown). None of the other investigated ILs were significantly degraded under these test conditions, therefore they cannot be classified as “readily biodegradable”. The degradability of the anion was not tested since FeCl4ˉ is lacking a carbon source and ethyl sulfate is known to be readily biodegradable.4
Conclusions and outlook
Optimized procedures for product work-up and anion metathesis have been elaborated, allowing access to a series of 13 new alkyloxymorpholinium salts with minimal efforts. X-Ray crystallography showed that in all cases the morpholinium ring adopts the expected regular chair conformation, and the alkyloxy groups occupy axial positions. There are numerous interionic contacts shorter than the sum of van der Waals radii. Despite bearing intentionally weak N–O linkages, the new compounds represent thermally stable, yet biosequesterable heterocyclic ILs. The new alkoxylated and the classical dialkylmorpholinium sub-groups resemble each other in their advantageous ecotoxicological profiles. Supplementarily, the chemical degradation of the cationic morpholinium cores was demonstrated by selective removal of the alkoxy side chain under hydrogenolytic conditions to yield the corresponding PILs, namely 4-methylmorpholinium methyl sulfate (16) and ethyl sulfate 17, respectively. Such environmentally benign PILs16 are capable of rapid aquatic dissemination and counter ion scrambling, eased by neutralization / reprotonation equilibria. When characteristics of ILs are revisited for further application development, environmental aspects such as biological and chemical degradability will always remain of major relevance.47
EXPERIMENTAL
N-Methylmorpholine N-oxide hydrate (1) (BASF-quality) was kindly donated by Lenzing AG, Austria. All other chemicals were obtained from commercial sources. NMR spectra were recorded with a Bruker Avance DPX 300 spectrometer. IR spectra were obtained with a Nicolet 5700 FT instrument. High resolution mass spectra were measured with a Finnigan MAT 95 mass spectrometer. Viscosities were measured with a MCR 300 rheometer. X-Ray diffraction data were collected with an Oxford Diffraction Gemini-R Ultra or Nonius KappaCCD diffractometer using Mo-Kα (λ = 0.7107 Å) or Cu-Kα radiation (λ = 1.5418 Å). CCDC 1005926–1005936 contain the supplementary crystallographic data for this paper. These data can be obtained free of charge from The Cambridge Crystallographic Data Centre.
N-Methoxy-N-methylmorpholinium methyl sulfate (2): This compound (CAS-RN [1320340-65-5]) has been described previously.7
N-Ethoxy-N-methylmorpholinium ethyl sulfate (3): To a suspension of N-methylmorpholine N-oxide hydrate (1) (100 g, 0.65 mol) in MeCN (20 mL), diethyl sulfate (101 g, 0.65 mol) was slowly added under cooling with ice. Subsequently, the ice-bath was removed, and the solution was stirred for 24 h. The solvent was evaporated and the remaining yellow liquid dried in high vacuum (177 g, 100%). nD20 = 1.465 (subcooled melt); mp 96 °C; IR (neat) 766, 553 cm–1; 1H NMR (300 MHz, DMSO-d6) δ 1.11 (t, J = 7.1 Hz, 3H), 1.24 (t, J = 6.9 Hz, 3H), 3.54 (s, 3H), 3.75 (q, J = 7.1 Hz, 2H), 3.85–3.90 (m, 8H), 4.11 (q, J = 6.9 Hz, 2H); 13C NMR (75 MHz, DMSO-d6) δ 12.3, 15.1, 51.0, 60.5 (2C), 61.0 (2C), 63.1, 63.4; MS (FAB) m/z 146.12 (calcd 146.12 for C7H16NO2, [M]+).
N-Methoxy-N-methylmorpholinium tetrachloroferrate(III) (4): To a solution of methyl sulfate 2 (10.8 g, 44 mmol) in H2O (50 mL), hydrochloric acid (37%, 3.7 mL, 44 mmol) and iron(III) chloride hexahydrate (12.0 g, 44 mmol) was added. The solution was stirred for 30 min. After removal of the solvent the residue was recrystallized from acetone and dried in high vacuum yielding 10.5 g (72%) of yellow crystals, mp 146 °C; IR (neat) 1117, 987, 865 cm–1; NMR spectra of this paramagnetic compound are depicted in the Supporting Information; MS (FAB) m/z 132.10 (calcd 132.10 for C6H14NO2, [M]+). Single-crystal diffraction: Gemini Ultra diffractometer, Mo-Kα radiation; ω scans; T = 133(2) K; θmax = 25.3°; indices: –8 ≤ h ≤ 8, –11 ≤ k ≤ 12, –19 ≤ l ≤ 21; Dx = 1.65 g cm–3; 7815 reflections measured, 2421 independent with Rint = 0.04, F(000) = 668, µ = 1.92 mm–1. R1 = 0.029 and wR2 = 0.058 for 2060 reflections with I>2σ(I), R1 = 0.038 and wR2 = 0.062 for all data; S = 1.03; Δρmax = 0.35 and Δρmin = –0.31 e Å–3. Crystal data for C6H14NO2·Cl4Fe (M = 329.83 g mol–1): monoclinic, P21/c, a = 7.4690(2), b = 10.0649(3), c = 17.7066(5) Å, β = 94.001(3), V = 1327.84(7) Å3, Z = 4. CCDC reference number: 1005926.
N-Ethoxy-N-methylmorpholinium tetrachloroferrate(III) (5): To a solution of ethyl sulfate 3 (21.2 g, 78 mmol) in H2O (80 mL), iron(III) chloride hexahydrate (21.1 g, 78 mmol) and hydrochloric acid (37%, 6.5 mL, 78 mmol) was added. The solution was stirred for 30 min. After removal of the solvent the residue was recrystallized from acetone and dried in high vacuum yielding 20.7 g (77%) of yellow crystals, mp 91 °C; IR (neat) 1108, 1001, 978, 864 cm–1; NMR spectra of this paramagnetic compound are depicted in the Supporting Information; MS (FAB) m/z 146.12 (calcd 146.12 for C7H16NO2, [M]+). Single-crystal diffraction: Gemini Ultra diffractometer, Mo-Kα radiation; ω scans; T = 133(2) K; θmax = 25.4°; indices: –9 ≤ h ≤ 7, –12 ≤ k ≤ 9, –9 ≤ l ≤ 11; Dx = 1.60 g cm–3; 4321 reflections measured, 2311 independent with Rint = 0.036, F(000) = 350, µ = 1.79 mm–1. R1 = 0.031 and wR2 = 0.066 for 2176 reflections with I>2σ(I), R1 = 0.035 and wR2 = 0.070 for all data; S = 1.07; Δρmax = 0.32 and Δρmin = –0.36 e Å–3. Crystal data for C7H16NO2·Cl4Fe (M = 343.86 g mol–1): monoclinic, P21, a = 7.4820(3), b = 10.2834(4), c = 9.6073(5) Å, β = 105.257(5)°, V = 713.14(5) Å3, Z = 2. CCDC reference number: 1005927.
N-Methoxy-N-methylmorpholinium chloride (6): Tetrachloroferrate 4 (1.0 g, 3.0 mmol) was dissolved in H2O (25 mL) and treated with ammonia (25% aqueous solution, 1.1 mL, 15 mmol). The resulting suspension was stirred overnight, the precipitate filtered off, and the filtrate was evaporated under reduced pressure. The residue was suspended in MeCN and filtered. The filtrate was evaporated to yield 430 mg (85%) as a white solid, mp 108–111 °C; IR (neat) 1105, 1007, 863 cm–1; 1H NMR (300 MHz, DMSO-d6) δ 3.53 (s, 3H), 3.84 (s, 3H), 3.88–3.94 (m, 8H); 13C NMR (75 MHz, DMSO-d6) δ 50.1, 54.9, 60.5 (4C); MS (FAB) m/z 132.11 (calcd 132.10 for C6H14NO2, [M]+). Single-crystal diffraction: Nonius KappaCCD diffractometer, Mo-Kα radiation; φ and ω scans; T = 233(2) K; θmax = 25.0°; indices: –6 ≤ h ≤ 5, –15 ≤ k ≤ 15, –13 ≤ l ≤ 11; Dx = 1.34 g cm–3; 2040 reflections measured, 1158 independent with Rint = 0.015, F(000) = 360, µ = 0.40 mm–1. R1 = 0.024 and wR2 = 0.064 for 1146 reflections with I>2σ(I), R1 = 0.024 and wR2 = 0.064 for all data; S = 1.07; Δρmax = 0.15 and Δρmin = –0.17 e Å–3. Crystal data for C6H14NO2·Cl (M = 167.63 g mol–1): monoclinic, Cc, a = 5.6130(3), b = 13.1310(3), c = 11.4215(6) Å, β = 98.773(2)°, V = 831.96(7) Å3, Z = 4. CCDC reference number: 1005928.
N-Ethoxy-N-methylmorpholinium chloride (7): The anhydrous chloride was serendipitously crystallized from MeCN solution. NMR data were identical to those of the hydrate. Single-crystal diffraction: Gemini Ultra diffractometer, Mo-Kα radiation; ω scans; T = 173(2) K; θmax = 25.3°; indices: –8 ≤ h ≤ 8, –17 ≤ k ≤ 14, –11 ≤ l ≤ 10; Dx = 1.34 g cm–3; 5305 reflections measured, 1644 independent with Rint = 0.018, F(000) = 392, µ = 0.38 mm–1. R1 = 0.027 and wR2 = 0.067 for 1486 reflections with I>2σ(I), R1 = 0.030 and wR2 = 0.069 for all data; S = 1.02; Δρmax = 0.25 and Δρmin = –0.17 e Å–3. Crystal data for C7H16NO2·Cl (M = 181.66 g mol–1): monoclinic, P21/n, a = 7.2872(4), b = 14.3432(6), c = 9.2113(6) Å, β = 111.227(7)°, V = 897.46(9) Å3, Z = 4. CCDC reference number: 1005929.
N-Ethoxy-N-methylmorpholinium chloride monohydrate (7·H2O): Tetrachloroferrate 5 (15.8 g, 0.046 mol) was dissolved in H2O (120 mL) and treated with ammonia (25% aqueous solution, 17.3 mL, 0.230 mol) upon which an orange precipitate formed. The suspension was stirred overnight, the precipitate filtered off, and the filtrate was evaporated under reduced pressure. The residue was suspended in MeCN (40 mL) and filtered. The filtrate was evaporated, and the residue was suspended in acetone and stirred for 3 h. The resulting white precipitate was filtered off, washed with acetone and dried (4.08 g, 41%), mp 92 °C; IR (neat) 1105, 1007, 863 cm–1; 1H NMR (300 MHz, DMSO-d6) δ 1.22 (t, J = 6.9 Hz, 3H), 3.67 (s, 3H), 3.85–3.93 (m, 8H), 4.15 (q, J = 6.9 Hz, 2H); 13C NMR (75 MHz, DMSO-d6) δ 12.3, 51.0, 60.5 (2C), 61.0 (2C), 63.1; MS (FAB) m/z 146.12 (calcd 146.12 for C7H16NO2, [M]+). Single-crystal diffraction: Gemini Ultra diffractometer, Mo-Kα radiation; ω scans; T = 133(2) K; θmax = 25.3°; indices: –9 ≤ h ≤ 9, –8 ≤ k ≤ 12, –14 ≤ l ≤ 15; Dx = 1.33 g cm–3; 5982 reflections measured, 3573 independent with Rint = 0.028, F(000) = 432, µ = 0.36 mm–1. R1 = 0.054 and wR2 = 0.163 for 2516 reflections with I>2σ(I), R1 = 0.072 and wR2 = 0.183 for all data; S = 1.08; Δρmax = 0.74 and Δρmin = –0.43 e Å–3. Crystal data for C7H16NO2·Cl·H2O (M = 399.35 g mol–1): triclinic, P1, a = 8.2618(8), b = 10.0862(8), c = 13.0944(12) Å, α = 78.494(7)°, β = 81.588(8)°, γ = 68.908(8)°, V = 994.28(15) Å3, Z = 2. CCDC reference number: 1005930.
N-Methoxy-N-methylmorpholinium periodate (8): A solution of chloride 6 (0.10 g, 0.60 mmol) and NaIO4 (0.13 g, 0.60 mmol) in H2O (20 mL) was stirred for 2 h. After solvent removal, the residue was suspended in MeCN (20 mL). Insoluble material was removed by filtration. The filtrate was evaporated to give a colorless solid which was recrystallized from MeOH, mp 83.5 °C. Yield: 0.11 g (57%). IR (neat) 839 cm–1; 1H NMR (300 MHz, DMSO-d6) δ 3.51 (s, 3H), 3.84 (s, 3H), 3.87–3.94 (m, 8H); 13C NMR (75 MHz, DMSO-d6) δ 50.0, 54.8, 60.4 (4C); MS (FAB) m/z 132.11 (calcd 132.10 for C6H14NO2, [M]+). Single-crystal diffraction: Gemini Ultra diffractometer, Mo-Kα radiation; ω scans; T = 173(2) K; θmax = 25.4°; indices: –5 ≤ h ≤ 8, –16 ≤ k ≤ 21, –8 ≤ l ≤ 10; Dx = 2.05 g cm–3; 6309 reflections measured, 1909 independent with Rint = 0.030, F(000) = 632, µ = 3.06 mm–1. R1 = 0.022 and wR2 = 0.047 for 1741 reflections with I>2σ(I), R1 = 0.025 and wR2 = 0.048 for all data; S = 1.06; Δρmax = 0.68 and Δρmin = –0.52 e Å–3. Crystal data for C6H14NO2·IO4 (M = 323.08 g mol–1): monoclinic, P21/c, a = 6.9543(3), b = 17.8954(7), c = 8.4838(3) Å, β = 96.249(4)°, V = 1049.54(7) Å3, Z = 4. CCDC reference number: 1005931.
N-Ethoxy-N-methylmorpholinium periodate (9): A solution of chloride 7 monohydrate (0.22 g, 1.1 mmol) and NaIO4 (0.24 g, 1.1 mmol) in H2O (40 mL) was stirred for 30 min and evaporated. The residue was suspended in MeCN (20 mL). Insoluble material was removed by filtration. The solution was evaporated ending up in a colorless, viscous liquid, which was dried in high vacuum leading to solid product. The product was recrystallized from MeOH yielding colorless crystals (0.19 g, 51%); mp 102–109 °C; IR (neat) 837 cm–1; 1H NMR (300 MHz, DMSO-d6) δ 1.37 (t, J = 6.9 Hz, 3H), 3.58 (s, 3H), 3.93 (m, 8H), 4.17 (q, J = 6.9 Hz, 2H); 13C NMR (75 MHz, DMSO-d6) δ 12.9, 52.4, 62.3 (2C), 63.2 (2C), 65.1; MS (FAB) m/z 146.12 (calcd 146.12 for C7H16NO2, [M]+). Single-crystal diffraction: Nonius KappaCCD diffractometer, Mo-Kα radiation; φ and ω scans; T = 233(2) K; θmax = 25.0°; indices: –12 ≤ h ≤ 12, –12 ≤ k ≤ 12, –16 ≤ l ≤ 16; Dx = 1.89 g cm–3; 9347 reflections measured, 2684 independent with Rint = 0.023, F(000) = 664, µ = 2.71 mm–1. R1 = 0.023 and wR2 = 0.055 for 2487 reflections with I>2σ(I), R1 = 0.025 and wR2 = 0.056 for all data; S = 1.06; Δρmax = 0.81 and Δρmin = –0.90 e Å–3. Crystal data for C7H16NO2·IO4 (M = 337.11 g mol–1): monoclinic, P21/n, a = 9.6497(2), b = 9.9159(2), c = 12.4990(3) Å, β = 97.916(1)°, V = 1184.58(4) Å3, Z = 4. CCDC reference number: 1005932.
N-Ethoxy-N-methylmorpholinium tetrafluoroborate (10): A mixture of chloride 7 monohydrate (0.22 g, 1.1 mmol) and NH4BF4 (0.12 g, 1.1 mmol) in MeCN (20 mL) was stirred for 1 h and filtered. The filtrate was taken to dryness, and the residue was recrystallized from CH2Cl2 to yield colorless needles (0.11 g, 43%). mp 102 °C; IR (neat) 1027, 1006 cm–1; 1H NMR (300 MHz, DMSO-d6) δ 1.25 (t, J = 6.9 Hz, 3H), 3.53 (s, 3H), 3.82–3.90 (m, 8H), 4.10 (q, J = 6.9 Hz, 2H); 13C NMR (75 MHz, DMSO-d6) δ 12.3, 51.0, 60.5 (2C), 61.0 (2C), 63.1; MS (FAB) m/z 146.12 (calcd 146.12 for C7H16NO2, [M]+). Single-crystal diffraction: Gemini Ultra diffractometer, Cu-Kα radiation; ω scans; T = 173(2) K; θmax = 67.5°; indices: –8 ≤ h ≤ 8, –28 ≤ k ≤ 24, –15 ≤ l ≤ 15; Dx = 1.41 g cm–3; 14198 reflections measured, 3966 independent with Rint = 0.033, F(000) = 976, µ = 1.26 mm–1. R1 = 0.055 and wR2 = 0.158 for 3330 reflections with I>2σ(I), R1 = 0.063 and wR2 = 0.168 for all data; S = 1.06; Δρmax = 0.50 and Δρmin = –0.34 e Å–3. Crystal data for C7H16NO2·BF4 (M = 233.02 g mol–1): monoclinic, P21/n, a = 7.0137(3), b = 23.6649(10), c = 13.3630(6) Å, β = 96.713(4)°, V = 2202.76(17) Å3, Z = 8. CCDC reference number: 1005933.
N-Ethoxy-N-methylmorpholinium azide (11): A mixture of chloride 7 monohydrate (0.22 g, 1.1 mmol) and NaN3 (72 mg, 1.1 mmol) in MeCN (20 mL) was stirred for 1 h and filtered. The filtrate was taken to dryness to give a brown oil. The product was dried in high vacuum leading to the formation of amber crystals (158 mg, 76%). mp 37–39 °C (decomposition); IR (neat) 2002 cm–1; 1H NMR (300 MHz, DMSO-d6) δ 1.25 (t, J = 6.9 Hz, 3H), 3.55 (s, 3H), 3.87–3.91 (m, 8H), 4.11 (q, J = 6.9 Hz, 2H); 13C NMR (75 MHz, DMSO-d6) δ 12.4, 51.0, 60.5 (2C), 61.0 (2C), 63.1; MS (FAB) m/z 146.12 (calcd 146.12 for C7H16NO2, [M]+). Single-crystal diffraction: Nonius KappaCCD diffractometer, Mo-Kα radiation; φ and ω scans; T = 233(2) K; θmax = 25.0°; indices: –12 ≤ h ≤ 13, –8 ≤ k ≤ 8, –13 ≤ l ≤ 14; Dx = 1.28 g cm–3; 4692 reflections measured, 1613 independent with Rint = 0.047, F(000) = 408, µ = 0.10 mm–1. R1 = 0.038 and wR2 = 0.097 for 1496 reflections with I>2σ(I), R1 = 0.042 and wR2 = 0.100 for all data; S = 1.07; Δρmax = 0.15 and Δρmin = –0.12 e Å–3. Crystal data for C7H16NO2·N3 (M = 188.24 g mol–1): orthorhombic, Pna21, a = 11.264(1), b = 7.122(1), c = 12.184(1) Å, V = 977.43(18) Å3, Z = 4. CCDC reference number: 1005934.
N-Ethoxy-N-methylmorpholinium acetate (12): Chloride 7 hydrate (200 mg, 1.0 mmol) was suspended in MeCN (10 mL) and sodium acetate trihydrate (136 mg, 1.0 mmol) was added. The mixture was stirred for an additional 2 h, the precipitate was filtered off, and the solvent was evaporated under reduced pressure. The residue was resuspended in acetone (10 mL) and filtered off. The volatiles were removed by means of a rotary evaporator, and drying (1 mbar) for 1 h gave the product as a brown liquid (100 mg, 49%). nD20 = 1.477; no phase transition was observed above –20 °C; IR (neat) 833 cm–1; 1H NMR (300 MHz, DMSO-d6) δ 1.25 (t, J = 6.9 Hz, 3H), 1.90 (s, 3H), 3.55 (s, 3H), 3.86–3.91 (m, 8H), 4.11 (q, J = 6.9 Hz, 2H); 13C NMR (75 MHz, DMSO-d6) δ 12.3, 21.2, 51.0, 60.5 (2C), 61.0 (2C), 63.1, 172.1; MS (FAB) m/z 146.12 (calcd 146.12 for C7H16NO2, [M]+). Dynamic viscosity (20 °C) η = 105 mPa s.
N-Ethoxy-N-methylmorpholinium dimethyl phosphate (13): A mixture of chloride 7 monohydrate (0.33 g, 1.7 mmol) and sodium dimethyl phosphate (0.25 g, 1.7 mmol) in MeCN (25 mL) was stirred for 1 h and filtered. The filtrate was taken to dryness to give a yellowish oil which was dried in high vacuum (0.19 g, 42%). nD20 = 1.445; no phase transition was observed above –20 °C; IR (neat) 1007, 864, 483 cm–1; 1H NMR (300 MHz, DMSO-d6) δ 1.23 (t, J = 6.9 Hz, 3H), 3.35 (d, J = 10.7 Hz, 3H), 3.59 (s, 3H), 3.82–3.93 (m, 8H), 4.13 (q, J = 6.9 Hz, 3H); 13C NMR (75 MHz, DMSO-d6) δ 12.4, 51.1, 51.9 (d, J = 5.7 Hz), 60.6 (2C), 61.1 (2C), 63.2; MS (FAB) m/z 146.12 (calcd 146.12 for C7H16NO2, [M]+). Dynamic viscosity (20 °C) η = 714 mPa s.
N-Ethoxy-N-methylmorpholinium hexafluorophosphate (14): Ethyl sulfate 3 (0.87 g, 3.2 mmol) was dissolved in H2O (10 mL), NH4PF6 (0.52 g, 3.2 mmol) was added, and the resulting white precipitate was filtered and washed with cold H2O (5 mL). Vacuum-drying for 3 h gave a white solid (0.64 g, 69%). Single crystals were grown by diffusion of Et2O into a CH2Cl2 solution at room temperature; mp 91 °C; IR (neat) 820, 554 cm–1; 1H NMR (300 MHz, DMSO-d6) δ 1.25 (t, J = 6.9 Hz, 3H), 3.53 (s, 3H), 3.79–3.92 (m, 8H), 4.10 (q, J = 6.9 Hz, 2H); 13C NMR (75 MHz, DMSO-d6) δ 12.2, 50.9, 60.4 (2C), 60.9 (2C), 63.0; MS (FAB) m/z 146.12 (calcd 146.12 for C7H16NO2, [M]+). Single-crystal diffraction: Gemini Ultra diffractometer, Cu-Kα radiation; ω scans; T = 133(2) K; θmax = 67.5°; indices: –10 ≤ h ≤ 10, –16 ≤ k ≤ 10, –12 ≤ l ≤ 12; Dx = 1.61 g cm–3; 11593 reflections measured, 2158 independent with Rint = 0.045, F(000) = 600, µ = 2.76 mm–1. R1 = 0.039 and wR2 = 0.099 for 1983 reflections with I>2σ(I), R1 = 0.042 and wR2 = 0.102 for all data; S = 1.09; Δρmax = 0.35 and Δρmin = –0.44 e Å–3. Crystal data for C7H16NO2·F6P (M = 291.18 g mol–1): monoclinic, P21/n, a = 8.6207(2), b = 13.5766(3), c = 10.4119(2) Å, β = 99.697(2)°, V = 1201.20(4) Å3, Z = 4. CCDC reference number: 1005935.
N-Ethoxy-N-methylmorpholinium triflimide (15): Ethyl sulfate 3 (9.7 g, 0.036 mol) was dissolved in H2O/CH2Cl2 (50 mL/50 mL), HCl (37%, 3.0 mL, 0.04 mol) and LiNTf2 (10.2 g, 0.036 mol) were added. The resulting mixture was extracted with CH2Cl2 (2 × 25 mL), the combined organic phases were washed with H2O (2 × 50 mL) and dried with Na2SO4. The solvent was removed under reduced pressure, and vacuum-drying for 3 h gave an orange liquid, which crystallized in the fridge (10.5 g, 69%), mp 28 °C. IR (neat) 1188, 1167, 1047, 612 cm–1; 1H NMR (300 MHz, DMSO-d6) δ 1.25 (t, J = 6.9 Hz, 3H), 3.53 (s, 3H), 3.85–3.90 (m, 8H), 4.10 (q, J = 6.9 Hz, 3H); 13C NMR (75 MHz, DMSO-d6) δ 12.1, 50.9, 60.4 (2C), 61.0 (2C), 63.0, 119.4 (q, J = 322 Hz, 2C); MS (FAB) m/z 146.12 (calcd 146.12 for C7H16NO2, [M]+). Single-crystal diffraction: Gemini Ultra diffractometer, Mo-Kα radiation; ω scans; T = 173(2) K; θmax = 25.4°; indices: –16 ≤ h ≤ 13, –8 ≤ k ≤ 8, –15 ≤ l ≤ 22; Dx = 1.66 g cm–3; 10683 reflections measured, 3133 independent with Rint = 0.027, F(000) = 872, µ = 0.40 mm–1. R1 = 0.035 and wR2 = 0.083 for 2678 reflections with I>2σ(I), R1 = 0.043 and wR2 = 0.088 for all data; S = 1.04; Δρmax = 0.32 and Δρmin = –0.36 e Å–3. Crystal data for C7H16NO2·C2F6NO4S2 (M = 426.36 g mol–1): monoclinic, P21/c, a = 13.5133(4), b = 7.0313(3), c = 18.2883(8) Å, β = 100.108(3)°, V = 1710.71(12) Å3, Z = 4. CCDC reference number: 1005936.
N-Methylmorpholinium methyl sulfate (16): A solution of 2 (8.0 g, 33 mmol) in MeOH (42 mL) was hydrogenated at 20 °C / 3 bar for 30 min using 5% Pd-C (0.5 g) as catalyst. The mixture was filtered and the solvent evaporated to give 5.7 g (81%) of the product as a clear colorless oil. nD20 = 1.461; no phase transition was observed above –20 °C; IR (neat) 1180, 889, 741 cm–1; 1H NMR (300 MHz, DMSO-d6) δ 2.82 (s, 3H), 3.40 (s, 3H), 3.10–3.90 (m, 8H), 9.52 (br s, 1H); 13C NMR (75 MHz, DMSO-d6) δ 42.6, 52.7 (2C), 53.1, 63.5 (2C) ; MS (FAB) m/z 102.09 (calcd 102.09 for C5H12NO, [M]+). Dynamic viscosity (20 °C) η = 267 mPa s.
N-Methylmorpholinium ethyl sulfate (17): A solution of 3 (4.00 g, 14.7 mmol) in H2O (20 mL) was hydrogenated at 20 °C / 3 bar for 50 min using 5% Pd-C (0.25 g) as catalyst. The mixture was filtered and the solvent evaporated to give 3.35 g (100%) of the product as a clear colorless oil. nD20 = 1.458; no phase transition was observed above –20 °C; IR (neat) 1178, 1006, 913 cm–1; 1H NMR (300 MHz, DMSO-d6) δ 1.11 (t, J = 7.1 Hz, 3H), 2.81 (d, J = 4.2 Hz, 3H), 3.01–3.12 (m, 2H), 3.36 (d, J = 12.5 Hz, 2H), 3.60 (Td, J = 12.9 and 1.9 Hz, 2H), 3.76 (q, J = 7.1 Hz, 2H), 3.96 (dd, J = 12.5 and 2.8 Hz, 2H), 9.56 (br s, 1H); 13C NMR (75 MHz, DMSO-d6) δ 15.1, 42.6, 52.7 (2C), 61.6, 63.5 (2C); MS (FAB) m/z 102.09 (calcd 102.09 for C5H12NO, [M]+). Dynamic viscosity (20 °C) η = 406 mPa s.
ACKNOWLEDGEMENTS
This work has been funded by the Tyrolean Science Fund within the project “Environmentally Benign Ionic Liquids for Technical Applications” (P. Nr. 172503). We also would like to thank the whole UFT team, especially Ulrike Bottin–Weber and Dr. Stephanie Steudte for lab work and valuable discussions. Dr. Doris E. Braun gratefully acknowledges funding by the Hertha Firnberg Program of the Austrian Science Fund (FWF, project T593-N19). We are grateful to Dr. Michael Hummel for viscosity measurements.
References
1. (a) N. V. Plechkova and K. R. Seddon, Chem. Soc. Rev., 2008, 37, 123; CrossRef (b) H. G. Joglekar, I. Rahman, and B. D. Kulkarni, Chem. Eng. Technol., 2007, 30, 819. CrossRef
2. N. Winterton, CHEManager Europe, May, 2008, 5.
3. M. Petkovic, K. R. Seddon, L. P. N. Rebelo, and C. Silva Pereira, Chem. Soc. Rev., 2011, 40, 1383. CrossRef
4. S. Stolte, S. Steudte, A. Igartua, and P. Stepnowski, Curr. Org. Chem., 2011, 15, 1946. CrossRef
5. T. Phuong T. Pham, C.-W. Cho, and Y.-S. Yun, Water Res., 2010, 44, 352.
6. Y. Deng, P. Besse-Hoggan, M. Sancelme, A.-M. Delort, P. Husson, and M. F. Costa Gomes, J. Hazard. Mater., 2011, 198, 165. CrossRef
7. M. Hummel, G. Laus, A. Schwärzler, G. Bentivoglio, E. Rubatscher, H. Kopacka, K. Wurst, V. Kahlenberg, T. Gelbrich, U. J. Griesser, T. Röder, H. K. Weber, H. Schottenberger, and H. Sixta, ACS Symp. Ser., 2010, 1033, 229. CrossRef
8. H. Sixta, M. Hummel, M. Iakovlev, and L. Tolonen, Int. Patent WO2013171364 A1, 2013.
9. W.-R. Pitner, J. Eichhorn, J. Von Hagen, P. A. Leland, and G. B. I. Scott, Int. Patent WO2009046840 A1, 2009.
10. G. Laus, A. Schwärzler, P. Schuster, G. Bentivoglio, M. Hummel, K. Wurst, V. Kahlenberg, T. Lörting, J. Schütz, P. Peringer, G. Bonn, G. Nauer, and H. Schottenberger, Z. Naturforsch., B: Chem. Sci., 2007, 62, 295.
11. C. Froschauer, R. Salchner, G. Laus, H. K. Weber, R. Tessadri, U. Griesser, K. Wurst, V. Kahlenberg, and H. Schottenberger, Aust. J. Chem., 2013, 66, 391. CrossRef
12. R. S. Boethling, E. Elizabeth, and D. DiFiore, Chem. Rev., 2007, 107, 2207. CrossRef
13. M. Ramdin, T. W. de Loos, and T. J. H. Vlugt, Ind. Eng. Chem. Res., 2012, 51, 8149. CrossRef
14. (a) Z.-Z. Yang, L.-N. He, Q.-W. Song, K.-H. Chen, A.-H. Liu, and X.-M. Liu, Phys. Chem. Chem. Phys., 2012, 14, 15832; CrossRef (b) C. Froschauer, H. K. Weber, T. Röder, H. Sixta, G. Laus, B. Lendl, and H. Schottenberger, Lenzinger Ber., 2013, 91, 30.
15. S. Viboud, N. Papaiconomou, A. Cortesi, G. Chatel, M. Draye, and D. Fontvieille, J. Hazard. Mater., 2012, 40, 216.
16. P. Brezana, J. Sierra, E. Martia, R. Cruanas, M. A. Garau, J. Arning, U. Bottin-Weber, and S. Stolte, J. Hazard. Mater., 2013, 261, 99. CrossRef
17. (a) J. Estager, J. D. Holbrey, and M. Swadzba-Kwasny, Chem. Soc. Rev., 2014, 43, 847; CrossRef (b) R. H. Herber, I. Nowik, M. E. Kostner, V. Kahlenberg, C. Kreutz, G. Laus, and H. Schottenberger, Int. J. Mol. Sci., 2011, 12, 6397. CrossRef
18. J. F. Fernandez, D. Waterkamp, and J. Thöming, Desalination, 2008, 224, 52. CrossRef
19. G. Meister and M. Wechsler, Biodegradation, 1998, 9, 91. CrossRef
20. W. M. Reichert, J. D. Holbrey, R. P. Swatloski, K. E. Gutowski, A. E. Visser, M. Nieuwenhuyzen, K. R. Seddon, and R. D. Rogers, Cryst. Growth Des., 2007, 7, 1106. CrossRef
21. W. A. Henderson, V. G. Jr. Young, P. Fylstra, H. C. De Long, and P. C. Trulove, Cryst. Growth Des., 2006, 6, 1645. CrossRef
22. F. F. Awwadi, R. D. Willett, K. A. Peterson, and B. Twamley, Chem. Eur. J., 2006, 12, 8952. CrossRef
23. E. R. Shepard and H. A. Shonle, J. Am. Chem. Soc., 1947, 69, 2269. CrossRef
24. (a) H. A. Shonle and E. R. Shephard, U.S. Patent US2493321, 1950; (b) L. P. Kyrides, U.S. Patent US2404299, 1946; (c) Monsanto Chemical Company, Brit. Patent GB626475, 1949.
25. F. Postleb, D. Stefanik, H. Seifert, and R. Giernoth, Z. Naturforsch., 2013, 68b, 1123.
26. K. S. Egorova and V. P. Ananikov, ChemSusChem, 2014, 7, 336. CrossRef
27. R. Biczak, B. Pawłowska, P. Bałczewski, and P. Rychter, J. Hazard. Mater., 2014, 274, 181. CrossRef
28. S. Steudte, P. Stepnowski, C.-W. Cho, J. Thöming, and S. Stolte, Chem. Commun., 2012, 48, 9382. CrossRef
29. (a) G. Laus, G. Bentivoglio,V. Kahlenberg, K. Wurst, G. Nauer, H. Schottenberger, M. Tanaka, H.-U. Siehl, Crystal Growth Des., 2012, 12, 1838; CrossRef (b) G. Bentivoglio, R. Krendelsberger, H.-P. Martinz, G. Nauer, W. Porcham, M. Rauch, H. Schottenberger, and G. Winkler, Int. Patent WO2006053362, 2006.
30. (a) C. Froschauer, H. Sixta, H. K. Weber, G. Laus, V. Kahlenberg, and H. Schottenberger, Chem. Lett., 2012, 41, 945; CrossRef (b) M. Hummel, C. Froschauer, G. Laus, T. Röder, H. Kopacka, L. K. J. Hauru, H. K. Weber, H. Sixta, and H. Schottenberger, Green Chem., 2011, 13, 2507; CrossRef (c) C. Froschauer, M. Hummel, G. Laus, H. Schottenberger, H. Sixta, H. K. Weber, and G. Zuckerstätter, Biomacromolecules, 2012, 13, 1973. CrossRef
31. (a) G. S. Groenewold, Main Group Chem., 2010, 9, 221; (b) Y.-C. Yang, Acc. Chem. Res., 1999, 32, 109. CrossRef
32. G. Quijano, A. Couvert, A. Amrane, G. Darracq, C. Couriol, P. Le Cloirec, L. Paquin, and D. Carrié, Chem. Eng. J., 2011, 174, 27. CrossRef
33. E. Ghanem, Y. Li, C. Xu, and F. M. Raushel, Biochemistry, 2007, 46, 9032. CrossRef
34. C. M. Da Costa Silva Pereira, L. P. N. Rebelo, and K. R. Seddon, Eur. Patent 1995305A1, 2008.
35. S. Gmorrissey and B. Pegot, Int. Patent WO2009024607 A1, 2009.
36. S. Stolte, M. Matzke, J. Arning, A. Böschen, W.-R. Pitner, U. Welz-Biermann, B. Jastorff, and J. Ranke, Green Chem., 2007, 9, 1170. CrossRef
37. J. S. Torrecilla, J. Palomar, J. Lemus, and F. Rodriguez, Green Chem., 2010, 12, 123. CrossRef
38. S. Stolte, J. Arning, U. Bottin-Weber, A. Müller, W.-R. Pitner, U. Welz-Biermann, B. Jastorff, and J. Ranke, Green Chem., 2007, 9, 760. CrossRef
39. C. Pretti, C. Chiappe, I. Baldetti, S. Brunini, G. Monni, and L. Intorre, Ecotoxicol. Environ. Saf., 2009, 72, 1170. CrossRef
40. J. Neumann, S. Steudte, C.-W. Cho, J. Thöming, and S. Stolte, Green Chem., 2014, 16, 2174. CrossRef
41. J. Arning, S. Stolte, A. Böschen, F. Stock, W.-R. Pitner, U. Welz-Biermann, B. Jastorff, and J. Ranke, Green Chem., 2008, 10, 47. CrossRef
42. M. Matzke, S. Stolte, K. Thiele, T. Juffernholz, J. Arning, J. Ranke, U. Welz-Biermann, and B. Jastorff, Green Chem., 2007, 9, 1198. CrossRef
43. S. Stolte, J. Arning, U. Bottin-Weber, M. Matzke, F. Stock, K. Thiele, M. Uerdingen, U. Welz-Biermann, B. Jastorff, and J. Ranke, Green Chem., 2006, 8, 621. CrossRef
44. S. Steudte, S. Bemowsky, M. Mahrova, U. Bottin-Weber, E. Tojo-Suarez, P. Stepnowski, and S. Stolte, RSC Adv., 2014, 4, 5198.
45. J. Ranke, K. Mölter, F. Stock, U. Bottin-Weber, J. Poczobutt, J. Hoffmann, B. Ondruschka, J. Filser, and B. Jastorff, Ecotoxicol. Environ. Saf., 2004, 58, 396. CrossRef
46. S. Stolte, S. Abdulkarim, J. Arning, A.-K. Blomeyer-Nienstedt, U. Bottin-Weber, M. Matzke, J. Ranke, B. Jastorff, and J. Thöming, Green Chem., 2008, 10, 214. CrossRef
47. R. Feng, D. Zhao, and Y. Guo, J. Environ. Prot., 2010, 1, 95. CrossRef