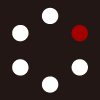
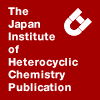
HETEROCYCLES
An International Journal for Reviews and Communications in Heterocyclic ChemistryWeb Edition ISSN: 1881-0942
Published online by The Japan Institute of Heterocyclic Chemistry
e-Journal
Full Text HTML
Received, 8th August, 2014, Accepted, 10th September, 2014, Published online, 18th September, 2014.
DOI: 10.3987/COM-14-S(K)106
■ Toward the Pluramycins: Route Exploration from Dihydroxyanthrone Tricyclic Platform to an Aglycon, Saptomycinone B
Kei Kitamura, Yoshio Ando, Yoshihiko Maezawa, Takashi Matsumoto,* and Keisuke Suzuki*
Depatrtment of Chemistry, Tokyo Institute of Technology, 2-12-1,Oh-Okayama, Meguro-ku, Tokyo 152-8552, Japan
Abstract
In connection with the total synthesis of the pluramycin-class antibiotics, we recently found dihydroxyanthrone derivatives to be suitable platforms for the installation of bis-C-glycosides. As a basis for the total synthesis, we explored viable routes to construct the characteristic tetracyclic skeleton. By setting saptomycinone B (5) as a model target, anthrone 6 was combined with the side chain moiety to access key intermediate 9, from which two viable routes have been developed. One of the routes has been exploited in the realization of our recent total synthesis of saptomycin B (4).INTRODUCTION
The pluramycins constitute a family of antibiotics featured by the polyketide-derived anthrapyranotrione chromophore containing two amino C-glycosides.1 Pluramycin A (1), reported in 1956 by Umezawa and Kondo et al., is the first member of this class, which was isolated from a terrestrial Streptomyces sp.2 Since then, many related compounds have appeared, including kidamycin (2),3 hedamycin (3),4 and saptomycin B (4)5 (Figure 1). The potent antitumor activity exhibited by several of these compounds has proven to be relevant to specific DNA alkylation, where the two amino sugars play a significant role in sequence recognition.6
In addition to such biological importance, the challenging structural features have attracted significant interests from the synthetic communities. Two major synthetic challenges include, (1) selective construction of the highly oxygenated tetracycle, and (2) installation of two C-glycosides, D-angolosamine at C8 and N,N-dimethyl-L-vancosamine at C10.
Initially described here are overviews of the previous aglycon synthesis,7 bis-C-glycoside synthesis8 and our own approaches.
Previous aglycon synthesis: Various strategies have emerged for the construction of the tetracyclic aglycon.7 Figure 2 shows a rough classification of these approaches by focusing on the last ring to be formed. The type-1 approach is featured by the formation of the pyranone (the A-ring) at the final stage onto the B/C/D tricyclic system. This approach has been applied to the syntheses of kidamycinone,7a,b espicufolin,7d indomycinones,7e–g and the related aglycons.7h–k Alternatively, the type-2 approach features the annulation of the D-ring onto the A/B/C ring system by Diels–Alder reaction.7c,l Unfortunately, these approaches are not strategically compatible with the installation of the bis-C-glycoside structure as stated below, and have not been exploited in the synthesis of the full structure of natural products.
Bis-C-glycoside synthesis: Studies toward the installation of the two C-glycosides have also been reported, which are roughly classified into two categories, early or late, by the timing of the bis-C-glycosylation (Figure 3).8 The early-stage approach uses mono- or bicyclic compounds as C-glycosyl acceptors. For example, we reported resorcinol derivative I as a monocyclic platform, enabling the installation of two sugars by repeated O→C glycoside rearrangement.8d Parker used naphthoquinone II as a bicyclic platform to accept two glycal anions followed by dienone–phenol rearrangement.8a Unfortunately, such approaches inevitably need long subsequent steps, and to date, no total syntheses using this strategy have been completed.
By contrast, the late-stage approach, if viable, is ideal in terms of its convergency and simplicity. However, the critical difficulty is the regiochemical control, e.g., McDonald used a pyranoanthracene IV as a glycosyl acceptor with an extra phenol to secure the regiochemical control and enhance the reactivity.8c Unfortunately, yields were low, and further conversion to the target has not been reported.
Martin employed a unique approach in his first synthesis of isokidamycin,9 through the C-glycosylation of a furan derivative followed by intramolecular benzyne cycloaddition and the late-stage introduction of the second sugar.
Our previous study and a turning point: Our synthetic endeavor toward the pluramycins had been unfruitful for a long time. Despite resorcinol derivative I (vide supra) allowing installation of the two requisite C-glycosides, the corresponding advanced intermediates were unexpectedly resistant to seemingly trivial transformations. After long struggle, we decided to explore any other timings for the bis-C-glycosylation. It occurred to us that the tricycle was a “missing ring” in the synthetic strategies explored to date, and indeed, this naive idea gave us a breakthrough.
Among the possible tricycles envisioned, we focused particularly on dihydroxyanthrones VII and VII’ as potential platform to install bis-C-glycoside by the following two-step deduction (Scheme 1). Starting from the intact B/C/D ring system V, we envisaged to remove the C7 carbonyl in a retrosynthetic fashion, leading to anthracene VI (step 1). This idea was inspired by the type-II polyketide biosynthesis (see A, Scheme 1),1,11 which, we hoped, would benefit us in two ways: (1) Anthracene VI, π-electron rich, would provide good opportunities for the installation of the two sugars by electrophilic aromatic substitutions (cf. quinone V). (2) This retrosynthetic strategy involves the late stage installation of the C7 carbonyl, which would help minimizing the possible photo-degradation associated with the pluramycins (see B, Scheme 1). 1a,12 Furthermore, the B-ring in VI was modified to non-aromatic as in VII and VII’ (step 2), expecting two additional benefits, (1) any undesired C-glycosylation at the B-ring could be avoided, and (2) the ketone could function as a handle to form the A-ring.
Pleasingly, tricycles VII and VII’ turned out to be excellent platforms for the installation of two sugars in complementary manners (Figure 4).10 Phenol VII allowed initial C-glycosylation to occur at C10 followed by C8. On the other hand, methyl ether VII’ enabled the first C-glycosylation at C8, and after demethylation, at C10. In each case, Sc(OTf)3 served as a specifically effective Lewis acid, enabling stepwise, regioselective C-glycosylations.13
With this promising basis, we re-launched the total synthesis, where prerequisite was to find the efficient conversion of tricyclic structures VII or VII’ into the complete tetracyclic structure. Since the chemical reactivities and selectivities are often different with/without the C-glycoside(s) as pointed out previously, the conversion should be truly efficient. We selected saptomycin B (4) as our first target, and accordingly explored the possible method of converting tricycle 6, through the union with synthon VIII, into its aglycon, saptomycinone B (5). Herein, we disclose the results of our investigation along these lines.
RESULTS AND DISCUSSION
As the initial step toward annulation of the A-ring, we connected the C4–C4a bond by aldol reaction (Scheme 2). Tricycle 614 was enolized (LDA, THF, –78 ºC, 0.5 h)14b and allowed to react with chiral, enantiopure (R)-aldehyde 7,15 giving aldol 8 in 97% yield as a diastereomeric mixture. Discovery of the most effective reagents and conditions for the subsequent oxidation of 8 required some experimentation: TPAP–NMO16 was ineffective, giving many unidentified products. Activated MnO2 led to slow reaction even at 80 ºC, giving only low yield of 9. Dess–Martin periodinane17 (CH2Cl2, 5 °C, 2 h) brought more success, albeit with the unsatisfactory yield of 9 (67%). Finally, IBX18 proved to be the reagent of choice. While the reaction in EtOAc (reflux, 11 h) gave 9 (28%) accompanied by pyranone 17 (29%), a mixed solvent (DMSO, CH2Cl2) brought about smooth reaction to give 1,3-diketone 9 in 87% yield. Note that the major tautomeric form of 9 is depicted.
At this stage, many possibilities were present en route to 5, differing in the order of performing three missions outlined below (Figure 6). Let us designate the shorthand for our missions as A, B, C; construction of the A-ring (Mission A), aromatization of the B-ring (Mission B), and oxygenation of the C-ring (Mission C).
Route 1 (Mission BAC): To explore the feasibility of the first B-ring aromatization, diketone 9 was acetylated to give 10 (Scheme 3). Oxidation of 10 with DDQ (benzene, reflux, 15 h) effected the dehydrogenation at the B-ring, giving anthracene 11 in 59% yield. At this stage, the A-ring cyclization was carried out under basic conditions (K2CO3, MeOH), where anthrapyranone 12 was obtained in 45% yield. The next task was removal of the acetyl group in 12, in preparation for the oxidation of the C-ring. However, basic hydrolysis of 12 caused decomposition exclusively under various conditions, and thus, this route was abandoned.
Route 2 (Mission CBA): Initial oxidation of the C-ring in 9 was attempted with PhI(OCOCF3)2 in aq. acetonitrile, which gave numerous products (TLC assay). In anhydrous methanol, however, the reaction gave the quinone acetal 14 in 39% yield. It should be noted that the B-ring underwent aromatization under these conditions. Subsequent A-ring cyclization of 14 was effected by Cs2CO3 (DMF, 0 °C, 40 min) to give 15 in 77% yield. For removal of the methyl-protecting group in 15, several Lewis acids were screened. While BBr3 only led to decomposition, MgI2·OEt219 nicely worked for the purpose to afford 16 in 62% yield. Finally, hydrolysis of the dimethyl acetal by TsOH (aq. THF, 60 °C, 4.5 h) gave the target 5 in 87% yield. The ee of 5 was assessed by chiral HPLC to be 98%, confirming that the overall conversion proceeded without loss of the stereochemical integrity of the C14 chiral center.
Route 3 (Mission ABC): Finally addressed was the prior cyclization of the A ring (Table 1). Organic bases, such as Et2NH and DMAP, gave γ-pyrone 17 in moderate yield (runs 1, 2). t-BuOK gave no desired product (run 3). In the case of Cs2CO3 in DMF, 17 was obtained in 23% yield (run 4). Screening of several polar solvents showed MeOH to be most effective, giving 17 in 85% yield (runs 5–7). In MeOH, K2CO3 was also effective, giving γ-pyrone 17 in 89% yield (run 8). In all runs, only the 6-endo cyclization was observed, and no furanone derived from the 5-exo-cyclization was detected.
Tetracycle 17 was oxidized by PhI(OCOCF3)2 in aq. acetonitrile20 (0 ºC, 20 min) to give naphthoquinone 18 in 80% yield (Scheme 5). Treatment of 18 with DBU (0 ºC, 30 min)21 followed by exposure to air gave anthraquinone 20 in 75% yield from 18. This conversion is rationalized by the following processes. DBU (pKa of the corresponding conjugate acid = 12) brought about the crisscross bis-enolization by abstracting the protons at C6 and then at C5, generating dihydroquinone 19, and its mono- and/or dianionic forms. Upon quenching and exposure to air, spontaneous oxidation gave pyranoanthraquinone 20. The final step was removal of the methyl protection, for which the combination of AlCl3 and n-Bu4NI (MeCN, reflux) was effective, giving the desired compound 5 in 63% yield. MgI2·OEt2 was even more effective, affording 5 in 92% yield (THF, reflux, 6 h). The enantiomeric excess of 5 was 97%, as assessed by chiral HPLC analysis. However, prolonged reaction caused partial epimerization of the C14 stereogenic center.
It is interesting to note an observation in this relevance. Upon treatment of naphthoquinone 18 with MgI2·OEt2 (toluene, 0 °C), anthraquinone 20 was observed on TLC. This suggested that a similar crisscross bis-enolization stated above occurred with of MgI2·OEt2, and the TLC spot of 20 was produced by aerial oxidation upon the spotting operation. Upon further stirring at higher temperature (80 ºC, 2.5 h), the demethylation proceeded in one-pot, giving aglycon 5 in 72% yield, which may provide a convenient protocol in total synthesis. At this moment, however, it has not been optimized and mechanistically unclear. The entity that undergoes the demethylation is unknown whether the magnesio derivative of hydroquinone 19 or quinone 20 derived from the unintentional contamination of oxygen.
CONCLUSION AND FUTURE OUTLOOK
We have described the development of two viable routes to convert anthrone 6 into saptomycinone B (5), differing in the order of elaboration of A, B, C-rings. One of these routes has secured as basis for our recent total synthesis of saptomycin B (4).15 Finally, it is worth noting further challenges in these tough synthesis. The first is the high lability of the natural products toward light and oxygen: we really had a hard time in the final stages of the synthesis of 4. Although basic protocols were established for purification and isolation, still great precaution must be paid.1a,12 The last challenging issue is the α-selective construction of vancosamine C-glycoside, which is the less favorable anomer, easily undergoing isomerization into the favorable β-anomer.1a,3c Further work is ongoing, seeking for the general synthesis of the pluramycins, including members bearing α-C-glycoside linkage.
ACKNOWLEDGMENTS
This work was supported by a Grant-in-Aid for Specially Promoted Research (No. 23000006) from Japan Society for the Promotion of Science (JSPS). We are grateful to Ms. Mhairi Matheson for careful proofreading.
EXPERIMENTAL
General. Melting points were measured on a Yanako MP-500 instrument. Optical rotations were determined on a Jasco DIP-1000 polarimeter. Infrared spectra were recorded on a Jasco IR-Report-100, or a Perkin Elmer Spectrum 100 spectrometer. Attenuated Total Reflectance Fourier Transform Infrared (ATR-FTIR) spectra were recorded on a Perkin Elmer 100 spectrometer. 1H and 13C NMR spectra were measured on a Bruker Avance III 600 (600 MHz) spectrometer, and chemical shifts (δ) are expressed in ppm using tetramethysilane as an internal standard (TMS = 0.0 ppm). Elemental analyses were recorded on an Elementar vario MICRO cube analyzer. High performance liquid chromatography analyses were performed using a Jasco CO-2060 apparatus. Low-resolution mass spectra (MS) were obtained with Shimadzu GCMS-QP 5050A spectrometer, and high-resolution MS from micrOTOF-Q II (Bruker Daltonics). Column chromatography was performed using silica gel 60N (Spherical, neutral, 63–210 µm; Kanto Chemical). Ethereal solvents (anhydrous; Kanto Chem. Co., Inc.) were used as received. Dichloromethane, acetonitrile, methanol, and DMSO were distilled prior to use by standard protocols. Other reagents were used without further purification as received.
9-Hydroxy-2-[(R,Z)-1-hydroxy-4-methyloct-6-en-2-yn-1-yliden]-8-methoxy-3-methyl-3,4-
dihydroanthracen-1(2H)-one (9): To a THF solution of LDA (0.156 M, 34.0 mL, 5.30 mmol) was added anthrone 614 (546 mg, 2.13 mmol) in THF (15 mL) at –78 ºC. After stirring at 0 ºC for 1 h, the mixture was re-chilled to –78 ºC, to which was slowly added aldehyde 7 (867 mg, 6.37 mmol) in THF (20 mL), and the stirring was continued for 30 min. After quenching by adding sat. NH4Cl soln, the products were extracted with EtOAc (×3). The combined organic extracts were washed with brine, dried (Na2SO4), and concentrated in vacuo. Purification by flash column chromatography (silica gel, hexane/acetone = 8/2) gave aldol 8 (812 mg, 97%, diastereomers) as yellow solids. Aldol 8 was dissolved in DMSO (18 mL) and CH2Cl2 (36 mL) and chilled to 0 ºC, to which was added IBX (1.19 g, 4.25 mmol). After stirring (2.5 h, room temp.), the reaction was stopped by adding sat. aq. NaHCO3. After extraction with EtOAc (×3), the combined organic extracts were washed with brine, 10% aq. Na2S2O3 and brine, and dried (Na2SO4). Concentration in vacuo and purification (SiO2 column chromatography, hexane/acetone = 9/1) gave ketone 9 (700 mg, 87%) as an orange oil: IR (neat) 2959, 2931, 2222, 1627, 1583, 1554, 1448, 1395, 1321, 1263, 1245, 1167, 1098, 841 cm–1; 1H NMR (CDCl3, 600 MHz) δ 1.05 (d, 3H, J = 7.0 Hz), 1.29 (d, 1.5H, J = 7.0 Hz), 1.30 (d, 1.5H, J = 7.0 Hz), 1.65 (d, 3H, J = 6.8 Hz), 2.28–2.39 (m, 2H), 2.68 (brd, 1H, J = 15.0 Hz), 2.76–2.82 (m, 1H), 3.14 (dd, 1H, J = 15.0, 5.3 Hz), 3.21–3.27 (m, 1H), 4.01 (s, 3H), 5.46–5.52 (m, 1H), 5.59–5.65 (m, 1H), 6.80 (d, 1H, J = 7.9 Hz), 6.95 (s, 1H), 7.20 (d, 1H, J = 8.1 Hz), 7.45 (dd, 1H, J = 8.1, 7.9 Hz), 14.28 (s, 1H, OH), 14.46 (s, 1H, OH); 13C NMR (CDCl3, 150 MHz) δ 13.0, 19.7, 19.8, 20.15, 20.17, 27.09, 27.13, 30.0, 33.3, 35.7, 56.2, 75.2, 105.7, 106.2, 110.8, 115.4, 117.4, 117.9, 119.8, 126.5, 126.6, 126.7, 126.8, 130.8, 135.9, 140.3, 156.5, 159.7, 164.8, 192.4; HRMS (ESI-TOF) calcd for C25H27O4 ([M+H]+) m/z 391.1904, found m/z 391.1902; Anal. Calcd for C25H26O4: C, 76.90; H, 6.71. Found: C, 76.91; H, 6.78.
2-[(R,Z)-Hex-4-en-2-yl]-12-hydroxy-11-methoxy-5-methyl-5,6-dihydroanthra[1,2-b]pyran-4-one (17): To a soln of ketone 9 (288 mg, 0.738 mmol) in MeOH (26 mL) was added K2CO3 (413 mg, 2.99 mmol) at 0 ºC, and the mixture was stirred for 6.5 h at room temp. After quenching with 1 M aq. HCl, the products were extracted with EtOAc (×3). Combined organic extracts were washed with brine, and dried (Na2SO4). Concentration in vacuo and purification (SiO2 column chromatography, hexane/acetone = 7/3) gave pyranone 17 (257 mg, 89%) as a pale yellow oil: IR (neat) 3327, 2967, 1645, 1585, 1495, 1455, 1421, 1364, 1266, 1235, 1178, 1091, 980, 925, 854, 802, 752, 723, 634 cm–1; 1H NMR (CDCl3, 600 MHz) δ 0.99 (d, 3H, J = 7.0 Hz), 1.34 (d, 1.5H, J = 7.0 Hz), 1.36 (d, 1.5H, J = 7.0 Hz), 1.58 (d, 1.5H, J = 6.7 Hz), 1.61 (d, 1.5H, J = 6.7 Hz), 2.35–2.44 (m, 1H), 2.57–2.66 (m, 1H), 2.71–2.77 (m, 2H), 3.07–3.13 (m, 1H), 3.44–3.50 (m, 1H), 4.12 (s, 3H), 5.38–5.45 (m, 1H), 5.52–5.59 (m, 1H), 6.216 (s, 0.5H), 6.222 (s, 0.5H), 6.81 (d, 1H, J = 7.6 Hz), 7.13 (s, 1H), 7.33 (d, 1H, J = 8.1 Hz), 7.37 (dd, 1H, J = 8.1, 7.6 Hz), 10.35 (s, 1H, OH); 13C NMR (CDCl3, 150 MHz) δ 12.78, 12.80, 16.87, 16.93, 17.4, 17.7, 24.1, 24.2, 31.60, 31.62, 36.10, 36.11, 38.75, 38.82, 56.5, 104.7, 109.6, 111.68, 111.73, 114.7, 118.6, 118.7, 121.3, 125.9, 126.2, 126.3, 127.1, 127.7, 137.1, 137.4, 153.97, 154.00, 157.1, 159.1, 170.9, 178.6; HRMS (ESI-TOF) calcd for C25H27O4 ([M+H]+) m/z 391.1904, found m/z 391.1905; Anal. Calcd for C25H26O4: C, 76.90; H, 6.71. Found: C, 76.79; H, 6.41.
2-[(R,Z)-Hex-4-en-2-yl]-11-methoxy-5-methyl-5,6-dihydro-4H-anthra[1,2-b]pyran-4,7,12 trione (18):
To pyranone 17 (25.1 mg, 64.2 µmol) in MeCN (2 mL) and H2O (23 µL, 1.3 mmol) was added PhI(OCOCF3)2 (56.1 mg, 130 µmol) at 0 ºC. After stirring (20 min, 0 ºC), the reaction was quenched by adding sat. aq. NaHCO3. The products were extracted with CH2Cl2 (×3), and the combined organic extracts were washed with brine, and dried (Na2SO4). Removal of the solvents in vacuo and purification (SiO2 column chromatography, hexane/acetone = 8/2) gave quinone 18 (20.9 mg, 80%) as a yellow oil: IR (ATR) 2971, 2933, 1644, 1586, 1471, 1419, 1368, 1307, 1273, 1214, 1066, 954, 925, 861, 751 cm–1; 1H NMR (CDCl3, 600 MHz) δ 1.06 (d, 3H, J = 7.1 Hz), 1.32 (d, 1.5H, J = 7.0 Hz), 1.35 (d, 1.5H, J = 7.0 Hz), 1.60 (d, 1.5H, J = 7.1 Hz), 1.62 (d, 1.5H, J = 7.1 Hz), 2.33–2.43 (m, 1H), 2.52–2.64 (m, 2H), 2.74–2.82 (m, 1H), 3.13 (brd, 1H, J = 18.6 Hz), 3.43–3.49 (m, 1H), 4.02 (s, 3H), 5.36–5.42 (m, 1H), 5.53–5.60 (m, 1H), 6.22 (s, 1H), 7.33 (d, 1H, J = 8.3 Hz), 7.68 (dd, 1H, J = 8.3, 7.6 Hz), 7.75 (d, 1H, J = 7.6 Hz); 13C NMR (CDCl3, 150 MHz) δ 12.8, 12.9, 17.45, 17.47, 17.7, 22.8, 26.5, 31.59, 31.60, 38.5, 38.6, 56.7, 112.5, 112.6, 118.29, 118.30, 119.0, 121.0, 126.5, 126.6, 126.7, 126.8, 129.45, 129.46, 134.0, 134.4, 134.6, 143.5, 154.8, 159.5, 172.3, 172.4, 177.9, 179.7, 184.3; HRMS (ESI-TOF) calcd for C25H25O5 ([M+H]+) m/z 405.1697, found m/z 405.1698; Anal. Calcd for C25H24O5: C, 74.24; H, 5.98. Found: C, 74.00; H, 6.19.
2-[(R,Z)-Hex-4-en-2-yl]-11-methoxy-5-methyl-4H-anthra[1,2-b]pyran-4,7,12 trione (20): To quinone 18 (155 mg, 0.383 mmol) in THF (8 mL) was added DBU (287 µL, 1.92 mmol) at 0 ºC. After stirring (0.5 h, 0 ºC), the reaction was quenched by 1 M aq. HCl. The products were extracted with CH2Cl2 (×3), and the combined organic extracts were washed brine, and dried (Na2SO4). Concentration in vacuo and purification (SiO2 column chromatography, hexane/acetone = 8/2) gave anthraquinone 20 (116 mg, 75%) as orange powders: Mp 177.5–178.2 ºC; [α]D30 –6.2 (c 0.17, CHCl3); IR (ATR) 2973, 2928, 1670, 1646, 1587, 1464, 1443, 1381, 1329, 1302, 1278, 1225, 1188, 1074, 1038, 968, 949, 849, 751 cm–1; 1H NMR (CDCl3, 600 MHz) δ 1.43 (d, 3H, J = 6.9 Hz), 1.61 (d, 3H, J = 6.7 Hz), 2.50 (ddd, 1H, J = 14.3, 7.2, 7.0 Hz), 2.72 (ddd, 1H, J = 14.3, 7.1, 7.0 Hz), 2.90 (ddq, 1H, J = 7.0, 7.0, 6.9 Hz), 2.97 (s, 3H), 4.04 (s, 3H), 5.42 (ddd, 1H, J = 10.8, 7.2, 7.1 Hz), 5.55 (dq, 1H, J = 10.8, 6.7 Hz), 6.21 (s, 1H), 7.36 (d, 1H, J = 8.3 Hz), 7.69 (dd, 1H, J = 8.3, 7.6 Hz), 7.87 (d, 1H, J = 7.6 Hz), 7.91 (s, 1H); 13C NMR (CDCl3, 150 MHz) δ 12.8, 17.7, 23.9, 31.5, 38.7, 56.7, 110.8, 118.5, 119.3, 122.6, 123.6, 124.4, 126.4, 126.5, 126.8, 134.4, 134.5, 135.0, 147.2, 155.9, 159.6, 172.6, 179.6, 180.6, 183.2; LRMS (EI-MS) m/z calcd for C25H22O5 [M+] 402; found: 402, 348, 295 (base peak); HRMS (ESI-TOF) Calcd for C25H23O5 ([M+H]+) m/z 403.1540, found m/z 403.1549; Anal. Calcd for C25H22O5: C, 74.61; H, 5.51. Found: C, 74.56; H, 5.52.
Saptomycinone B (5): To a soln of anthraquione 20 (12.7 mg, 31.5 µmol) in THF (0.8 mL) was added MgI2·OEt2 (0.2 M in Et2O, 0.80 mL, 0.16 mmol) at 0 ºC. After stirring (6 h, 80 ºC), the reaction was quenched by adding sat. aq. NaHCO3. The products were extracted with EtOAc (×3), and the combined organic extracts were washed by brine, 10% aq. Na2S2O3, brine, and dried (Na2SO4). Removal of the solvents in vacuo and purification (SiO2 column chromatography, hexane/acetone = 85/15) gave 5 (11.3 mg, 92%) as orange powders: Mp 141–147 ºC (CHCl3/hexane); [α]D20 –24 (c 0.10, CHCl3); IR (ATR) 2975, 2926, 1674, 1648, 1578, 1458, 1448, 1374, 1315, 1259, 1207, 1159, 1083, 1011, 914, 839, 790, 750 cm–1; 1H NMR (CDCl3, 600 MHz) δ 1.44 (d, 3H, J = 6.8 Hz), 1.60 (dd, 3H, J = 6.8, 1.5 Hz), 2.51 (ddd, 1H, J = 14.3, 7.1, 7.0 Hz), 2.73 (ddd, 1H, J = 14.3, 7.2, 7.0 Hz), 2.86 (ddq, 1H, J = 7.0, 7.0, 6.8 Hz), 3.02 (s, 3H), 5.38–5.44 (m, 1H), 5.53–5.59 (m, 1H), 6.24 (s, 1H), 7.36 (dd, 1H, J = 8.3, 1.0 Hz), 7.67 (dd, 1H, J = 8.3, 7.5 Hz), 7.82 (dd, 1H, J = 7.5, 1.1 Hz), 8.05 (s, 1H), 12.94 (s, 1H, OH); 13C NMR (CDCl3, 150 MHz) δ 12.9, 17.6, 24.2, 31.6, 39.0, 111.2, 116.8, 119.3, 119.7, 125.3, 125.5, 126.5, 126.6, 126.7, 132.3, 135.9, 136.3, 149.7, 156.7, 162.6, 172.4, 179.2, 182.0, 187.2; UV (MeOH) λmax nm (ε) 240 (47000), 268 (24000), 417 (8000); LRMS (EI-MS) m/z calcd for C24H20O5 [M+] 388; found: 388, 334, 281 (base peak); HRMS (ESI-TOF) calcd for C24H21O5 ([M+H]+) m/z 389.1383, found m/z 389.1395; Anal. Calcd for C24H20O5: C, 74.21; H, 5.19. Found: C, 74.04; H, 5.47; HPLC [CHIRALPAK® AD-H (Daicel) φ 0.46×25 cm, hexane:isopropyl alcohol = 9:1, 1.0 mL/min, 30 ºC, 254 nm] tR: 9.0 min for 5 (10.8 min for ent-5).
References
1. (a) U. Séquin, Fortschr. Chem. Org. Naturst., 1986, 50, 57; CrossRef (b) T. Bililign, B. R. Griffith, and J. S. Thorson, Nat. Prod. Rep., 2005, 22, 742. CrossRef
2. (a) K. Maeda, T. Takeuchi, K. Nitta, K. Yagishita, R. Utahara, T. Osato, M. Ueda, S. Kondo, Y. Okami, and H. Umezawa, J. Antibiot. Ser. A, 1956, 9, 75; (b) S. Kondo, M. Miyamoto, H. Naganawa, T. Takeuchi, and H. Umezawa, J. Antibiot., 1977, 30, 1143; CrossRef (c) M. Ceroni and U. Séquin, Helv. Chim. Acta, 1982, 65, 302. CrossRef
3. (a) N. Kanda, J. Antibiot., 1971, 24, 599; CrossRef (b) M. Furukawa, A. Itai, and Y. Iitaka, Tetrahedron Lett., 1973, 14, 1065; CrossRef (c) M. Furukawa and Y. Iitaka, Tetrahedron Lett., 1974, 15, 3287. CrossRef
4. (a) U. Séquin, C. T. Bedford, S. K. Chung, and A. I. Scott, Helv. Chim. Acta, 1977, 60, 896; CrossRef (b) U. Séquin, Tetrahedron, 1978, 34, 761; CrossRef (c) M. Zehnder, U. Séquin, and H. Nadig, Helv. Chim. Acta, 1979, 62, 2525. CrossRef
5. (a) N. Abe, Y. Nakakita, T. Nakamura, N. Enoki, H. Uchida, and M. Munekata, J. Antibiot., 1993, 46, 1530; CrossRef (b) N. Abe, N. Enoki, Y. Nakakita, H. Uchida, T. Nakamura, and M. Munekata, J. Antibiot., 1993, 46, 1536. CrossRef
6. (a) M. R. Hansen and L. H. Hurley, Acc. Chem. Res., 1996, 29, 249; CrossRef (b) B. Willis and D. P. Arya, Curr. Org. Chem., 2006, 10, 663. CrossRef
7. kidamycinone: (a) F. M. Hauser and R. P. Rhee, J. Am. Chem. Soc., 1979, 101, 1628; CrossRef (b) Z.-B. Fei and F. E. McDonald, Org. Lett., 2005, 7, 3617; CrossRef espicufolin: (c) H. Uno, K. Sakamoto, E. Honda, and N. Ono, Chem. Commun., 1999, 1005; CrossRef (d) L. F. Tietze, K. M. Gericke, R. R. Singidi, and I. Schuberth, Org. Biomol. Chem., 2007, 5, 1191; CrossRef indomycinone: (e) D.-S. Hsu, T. Matsumoto, and K. Suzuki, Chem. Lett., 2006, 35, 1016; CrossRef (f) L. F. Tietze, R. R. Singidi, and K. M. Gericke, Org. Lett., 2006, 8, 5873; CrossRef (g) K. Krohn, H. T. Tran-Thien, and I. Ahmed, Eur. J. Org. Chem., 2011, 2223; CrossRef other related aglycons: (h) K. Krohn and J. Vitz, Eur. J. Org. Chem., 2004, 209; CrossRef (i) K. Krohn, H. T. Tran-Thien, J. Vitz, and A. Vidal, Eur. J. Org. Chem., 2007, 1905; (j) L. F. Tietze, R. R. Singidi, K. M. Gericke, H. Böckemeier, and H. Laatsch, Eur. J. Org. Chem., 2007, 5875; CrossRef (k) L. F. Tietze, R. R. Singidi, and K. M. Gericke, Chem. Eur. J., 2007, 13, 9939; CrossRef (l) B. J. D. Wright, J. Hartung, F. Peng, R. V. de Water, H. Liu, Q.-H. Tan, T.-C, Chou, and S. J. Danishefsky, J. Am. Chem. Soc., 2008, 130, 16786. CrossRef
8. (a) K. A. Parker and Y.-H. Koh, J. Am. Chem. Soc., 1994, 116, 11149; CrossRef (b) D. E. Kaelin, Jr., S. M. Sparks, H. R. Plake, and S. F. Martin, J. Am. Chem. Soc., 2003, 125, 12994; CrossRef (c) Z.-B. Fei and F. E. McDonald, Org. Lett., 2007, 9, 3547; CrossRef (d) M. Shigeta, T. Hakamata, Y. Watanabe, K. Kitamura, Y. Ando, K. Suzuki, and T. Matsumoto, Synlett, 2010, 2654.
9. (a) B. M. O’Keefe, D. M. Mans, D. E. Kaelin, Jr., and S. F. Martin, J. Am. Chem. Soc., 2010, 132, 15528; CrossRef (b) B. M. O’Keefe, D. M. Mans, D. E. Kaelin, Jr., and S. F. Martin, Tetrahedron, 2011, 67, 6524. CrossRef
10. K. Kitamura, Y. Ando, T. Matsumoto, and K. Suzuki, Angew. Chem. Int. Ed., 2014, 53, 1258. CrossRef
11. A. Das and C. Khosla, Acc. Chem. Res., 2009, 42, 631. CrossRef
12. (a) A. Fredenhagen and U. Séquin, Helv. Chim. Acta, 1985, 68, 391; CrossRef (b) A. Fredenhagen and U. Séquin, J. Antibiot., 1985, 38, 236. CrossRef
13. (a) S. Kobayashi, Eur. J. Org. Chem., 1999, 15; CrossRef (b) A. Ben, T. Yamauchi, T. Matsumoto, and K. Suzuki, Synlett, 2004, 225.
14. (a) F. J. Leeper and J. Stauton, J. Chem. Soc., Perkin Trans. 1, 1984, 1053; CrossRef (b) J. H. Dodd, J. E. Starrett, and S. M. Weinreb, J. Am. Chem. Soc., 1984, 106, 1811. CrossRef
15. K. Kitamura, Y. Maezawa, Y. Ando, T. Kusumi, T. Matsumoto, and K. Suzuki, Angew. Chem. Int. Ed., 2014, 53, 1262. CrossRef
16. S. V. Ley, J. Norman, W. P. Griffith, and S. P. Marsden, Synthesis, 1994, 639. CrossRef
17. D. B. Dess and J. C. Martin, J. Org. Chem., 1983, 48, 4155. CrossRef
18. (a) M. Frigerio, M. Santagostino, and S. Sputore, J. Org. Chem., 1999, 64, 4537; CrossRef (b) A. Duschek and S. F. Kirsch, Angew. Chem. Int. Ed., 2011, 50, 1524. CrossRef
19. (a) S. Yamaguchi, K. Sugiura, R. Fukuoka, K. Okazaki, M. Takeuchi, and Y. Kawase, Bull. Chem. Soc. Jpn., 1984, 57, 3607; CrossRef (b) S. A. Snyder, Z.-Y. Tang, and R. Guptav, J. Am. Chem. Soc., 2009, 131, 5744. CrossRef
20. (a) R. Barret and M. Daudon, Tetrahedron Lett., 1990, 31, 4871; CrossRef (b) V. V. Zhdankin and P. J. Stang, Chem. Rev., 2008, 108, 5299. CrossRef
21. (a) D. M. Gorden and S. J. Danishefsky, J. Org. Chem., 1992, 57, 7052; CrossRef (b) T. Matsumoto, T. Sohma, H. Yamaguchi, S. Kurata, and K. Suzuki, Tetrahedron, 1995, 51, 7347. CrossRef