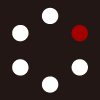
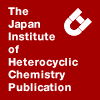
HETEROCYCLES
An International Journal for Reviews and Communications in Heterocyclic ChemistryWeb Edition ISSN: 1881-0942
Published online by The Japan Institute of Heterocyclic Chemistry
e-Journal
Full Text HTML
Received, 18th September, 2014, Accepted, 23rd October, 2014, Published online, 4th November, 2014.
DOI: 10.3987/COM-14-S(K)105
■ Theoretical Study on Radical Trifluoromethylation of Silyl Enol Ethers Accelerated via Complexation with Dialkylzinc
Susumu Kawauchi, Yoshihiro Hayashi, Yuichi Tomita, Ryota Hashimoto, Kazuya Honda, Yoshimitsu Itoh, and Koichi Mikami*
Department of Applied Chemistry, Graduate School of Science and Technology, Tokyo Institute of Technology, 2-12-1 Ookayama, Meguro-ku, Tokyo 152-8552, Japan
Abstract
Theoretical study on the electrophilic radical trifluoromethylation of silyl enol ethers is reported to give α-trifluoromethyl carbonyl compounds; the complexation with dialkylzinc accelerates the radical addition of highly electrophilic trifluoromethyl radical to silyl enol ethers via the Zn-π complex (A) rather than the Zn-O complex (B).Trifluoromethyl compounds have attracted current interest, because of their important applications as biologically active and physically intriguing compounds for medicinal, material, and life sciences.1 α-Trifluoromethyl carbonyl compounds are the most promising building blocks for the construction of these trifluoromethyl compounds. The addition of an electrophilic trifluoromethyl reagent to nucleophilic metal enolates is in principle one of the simplest ways to introduce a trifluoromethyl unit at the α position of the carbonyl compounds. However, polarization of trifluoromethyl iodide (CF3δ--Iδ+) is in the opposite way to that of methyl iodide (CH3δ+-Iδ-). Therefore, the reaction of metal enolates with trifluoromethyl iodide itself cannot provide α-trifluoromethyl carbonyl compounds.2 Limited examples have so far been reported even on radical trifluoromethylation with trifluoromethyl iodide of the metal enolates.3-6 The difficulty in the radical trifluoromethylation of nucleophilic metal enolates is due to defluorination of the α-trifluoromethyl carbonyl compounds by the parent metal enolates or bases employed under the reaction conditions (Scheme 1).4
Previously, we reported the use of titanium ate enolates7 and even lithium enolates8 could avoid significant defluorination by their rapid radical trifluoromethylation of the “metal enolates”. However, these methods are only effective with limited scope of carbonyl compounds. Less basic enolate equivalents such as silyl enol ethers have been used for radical trifluoromethylation to suppress defluorination of the α-trifluoromethyl carbonyl products.3 However, this silyl enol ether method can only be applied for ester silyl enol ethers (ketene silyl acetals), which are more nucleophilic than ketone silyl enol ethers of poor reactivity in radical trifluoromethylation. We thus attempted to use less basic zinc metal enolates;1d,9 The combination of soft metal zinc10 with hard fluorine suggests the negligible zinc-fluoride elimination even from the α-trifluoromethyl zinc enolates (Scheme 1).
The zinc enolates were thus examined to generate from ketone silyl enol ethers and dialkylzinc11 for radical trifluoromethylation. Although the reactivity of the trimethylsilyl enol ether is significantly increased for radical trifluoromethylation, the formation of the zinc enolate was not observed under the reaction conditions by NMR analyses. Furthermore, the use of dialkylzinc can be reduced in catalytic amount in the radical trifluoromethylation of silyl enol ethers (Scheme 2 and Table 1). As expected, even with a semi-catalytic amount of diethylzinc (0.5 equiv), the trifluoromethylation product was obtained in good yield (55%, 1 h; 76%, 20 h) (entries 6 and 7). In the absence of diethylzinc, the trifluoromethylation product was not obtained in sufficient yields (entries 1~3).3
Several substrates were investigated to give good-to-moderate yields of the α-trifluoromethyl carbonyl compounds (Scheme 3 and Table 2). The radical trifluoromethylation was found to give wide applicability of substrates including heterocyclic and steroidal compounds. The wide scope of substrates is in sharp contrast to titanium ate enolate,7 which is not well applicable to (steroidal) cyclopentanone (entries 7 vs. 8 and 9), and lithium enolate,7a,8 which is limited to cyclohexanone derivatives (entries 5 vs. 6 and 8). Acyclic substrates (entries 12 vs. 13 and 14 vs. 15) as well as cyclic substrates including heterocyclic compound (entry 16) provided the α-trifluoromethyl carbonyl products in good-to-moderate yields, via the silyl enol ethers activated with dialkylzinc.
These observations imply that the complexation of dialkylzincs with the silyl enol ethers might involve the 3d orbital of Zn/π∗-orbital of silyl enol ether complex (A) or the Lewis acidic zinc (4p vacant orbital)/Lewis basic oxygen (lone pair electrons) of silyl enol ether complex (B) as depicted in Figure 1.12 To clarify the mode (A or B) and role of the Zn-complexation for trifluoromethylation, the DFT calculations were carried out for trifluoromethylation and methylation of the model complexes between acetone silyl enol ether (1) and dimethylzinc (ZnMe2) using the ωB97X-D/TZVP level of theory.13 In Figure 2, the optimized structures of reactants, CH3 and CF3 radicals, 1, and ZnMe2, are collected. As shown in Figure 2, a carbon atom in CF3 radical is pyramidized with highly positive charge (+0.909 e), while a carbon atom in CH3 radical is planar with moderately negative charge (-0.490 e).
We considered the two possibilities as to whether the Zn-π complex A or the Zn-O complex B. The optimized geometries for these two complexes (A and B) are displayed in Figure 3. It is interesting to note that for the complex A, the Zn-C2 bond (3.249 Å) is longer than the Zn-C1 bond (2.930 Å). Compared with the optimized structures as shown in Figures 2 and 3, the geometrical perturbation of 1 and ZnMe2 after complexation with ZnMe2 is relatively small for both complexes. The calculated complexation energy for A and B is -7.48 kcal/mol and -8.19 kcal/mol, respectively (Table 3). From these results, it is clear that interactions between 1 and ZnMe2 for both complexes are thermodynamically favored but weak. If the interaction is strong, the “transmetalation” between SiMe3 moiety in 1 and ZnMe moiety in ZnMe2 to give zinc enolate (2) should be facilitated. The transition state (TS1) for Si→Zn transmetalation was optimized (Figure 3); The calculated activation energy (Ea) is 41.97 kcal/mol and the heat of the transmetallation reaction (ΔH) is 11.49 kcal/mol endothermic (Table 3). Therefore, the transmetalation reaction is disfavored not only kinetically but also thermodynamically. This is consistent with the NMR experiment that the zinc enolate was not observed by the addition of ZnMe2 to the trimethylsilyl enol ether (1). The natural charges for selected atoms are also shown in Figures 2 and 3. It is interesting to note that the charge on C1 is -0.545 e for 1, -0.585 e for complex A, and -0.494 e for complex B, respectively. It is highly expected that the electrophilic radical trifluoromethylation on C1 will be accelerated via not the complex B but the complex A driven mainly by charge-controlled mechanism, while nucleophilic radical methylation will be in an opposite manner controlled by frontier-orbital interaction.
The optimized structures of the transition states (TSs) for the trifluoromethylation and methylation of complexes A and B are shown in Figure 4, respectively. The calculated activation energies (Ea) and heats of reaction (ΔH) are collected in Table 3. Ea for the trifluoromethylation of complex A (-1.86 kcal/mol) is smaller than that of complex B (-1.14 kcal/mol). This is ascribed to the more negative charge on C1 in complex A than that in complex B. The reason of minus values for the activation energy may be explained by instability of the bare trifluoromethyl radical and the electron spin delocalization in the TS's. Since charge separation is disfavored in gas phase, the activation energy for trifluoromethylation of complex A in condensed phase should be much smaller than that of complex B. Therefore, it seems reasonable to assume that the electrophilic radical trifluoromethylation on C1 must be accelerated through complex A, while the nucleophilic radical methylation on C1 may be accelerated through complex B. Complex A is thus proposed as the key for trifluoromethylation of highly electrophilic and pyramiderized trifluoromethyl radical (Figure 2). In a polar solvent, Zn-π complex A is likely formed in the presence of dialkylzinc and, hence, the addition of trifluoromethyl radical onto the C1 atom will be facilitated.
The results of Natural Bond Orbital (NBO) analysis13 for complex A and B are depicted in Figure 5. It is clear that the orbital interaction between the 4p vacant orbital of Zn and the π-orbital of silyl enol ether was the largest of 5.75 kcal/mol in complex A, but the next one was the interaction between the 3d orbital of Zn and the π*-orbital of silyl enol ether. This indicates that the orbital interaction between the 3d orbital of Zn and π*-orbital of silyl enol ether plays an additional role for acceleration of trifluoromethylation of silyl enol ethers by dialkylzinc.
In conclusion, we have thus developed the radical trifluoromethylation of silyl enol ethers accelerated by dialkylzinc complexation; Activation with dialkylzinc leads to the α-trifluoromethyl carbonyl compounds in increased yields with wide scope of the substrates including acyclic and cyclic ketones including cyclopentanonone, and heterocyclic compounds. Theoretical study shows that the Zn-π complex rather than the Zn-O complex plays the important role for trifluoromethylation of silyl enol ethers. The use of dialkylzinc to activate the silyl enol ethers is the key to the efficient radical trifluoromethylation, by which trifluoromethyl substituent can be introduced to give various α-trifluoromethyl carbonyl compounds without formation of the zinc enolate intermediates.
ACKNOWLEDGEMENTS
We are grateful to Tosoh F-Tech Inc. for their generous supply of trifluoromethyl iodide. All the theoretical calculations were carried out on the TSUBAME2.5 supercomputer at the Tokyo Institute of Technology, Tokyo, Japan, and on the supercomputer at the Research Center for Computational Science, Okazaki, Japan.
References
1. (a) P. Kirsch, Modern Fluoroorganic Chemistry, 2nd Ed., Wiley-VCH, Weinheim, 2013; CrossRef (b) I. Ojima, Ed., Fluorine in Medicinal Chemistry and Chemical Biology, Blackwell, Oxford, 2009; CrossRef (c) J.-P. Bérgué and D. Bonnet-Delpon, Bioorganic and Medicinal Chemistry of Fluorine, Wiley, Hoboken, 2008; CrossRef (d) K. Mikami, Y. Itoh, and M. Yamanaka, Chem. Rev., 2004, 104, 1; CrossRef (e) J. -A. Ma and D. Cahard, Chem. Rev., 2004, 104, 6119. CrossRef
2. (a) J. E. Huheey, J. Phys. Chem., 1965, 69, 3284; CrossRef (b) M. Yoshida and N. Kamigata, J. Fluorine Chem., 1990, 49, 1. CrossRef
3. Perfluoroalkylation of silyl and germyl enolates of esters and ketones: (a) K. Miura, M. Taniguchi, K. Nozaki, K. Oshima, and K. Utimto, Tetrahedron Lett., 1990, 31, 6391; CrossRef (b) K. Miura, Y. Takeyama, K. Oshima, and K. Utimoto, Bull. Chem. Soc. Jpn., 1991, 64, 1542; Perfluoroalkylation of silyl enol ethers provided the products in good yields except for trifluoromethylation. Trifluoromethylation of ketone germyl enolates proceeds in good yield. CrossRef
4. Trifluoromethylation of lithium enolate of imides: (a) K. Iseki, T. Nagai, and Y. Kobayashi, Tetrahedron Lett., 1993, 34, 2169; CrossRef (b) K. Iseki, T. Nagai, and Y. Kobayashi, Tetrahedron: Asymmetry, 1994, 5, 961; They have succeeded in trifluoromethylation by adopting Evans oxazolidinones with bulky substitutent at α position to suppress defluorination. CrossRef
5. (a) Recent review on radical trifluoromethylation: A. Studer, Angew. Chem. Int. Ed., 2012, 51, 2; CrossRef (b) Radical trifluoromethylation of enamines: D. Cantacuzène, C. Wakselman, and R. Dorme, J. Chem. Soc., Perkin Trans. 1, 1977, 1365; CrossRef (c) T. Kitazume and N. Ishikawa, J. Am. Chem. Soc., 1985, 107, 5186; CrossRef Asymmetric trifluoromethylation: (d) D. A. Nagib and D. W. C. MacMillan, Nature, 2011, 480, 224, and references cited therein. CrossRef
6. There are some reports of trifluoromethylation using CF3+: (a) N. Santschi and A. Togni, Chimia, 2014, 68, 419; CrossRef (b) L. M. Yagupol’skii, N. V. Kondratenko, and G. N. Timofeeva, J. Org. Chem. USSR, 1984, 20, 115; (c) T. Umemoto and K. Adachi, J. Org. Chem., 1994, 59, 5692, and references cited therein; CrossRef Radical trifluoromethylation via xanthate radical transfer: (d) F. Bertrand, V. Pevere, B. Quiclet-Sire, and S. Z. Zard, Org. Lett., 2001, 3, 1069. CrossRef
7. (a) Y. Itoh, K. N. Houk, and K. Mikami, J. Org. Chem., 2006, 71, 8918; CrossRef (b) Y. Itoh and K. Mikami, Org. Lett., 2005, 7, 649; CrossRef (c) Y. Itoh and K. Mikami, J. Fluorine Chem., 2006, 127, 539. CrossRef
8. (a) Y. Itoh and K. Mikami, Org. Lett., 2005, 7, 4883; CrossRef (b) Y. Itoh and K. Mikami, Tetrahedron, 2006, 62, 7199. CrossRef
9. (a) H. Plenio, Chem. Rev., 1997, 97, 3363; CrossRef (b) E. F. Murphy, R. Murugavel, and H. W. Roesky, Chem. Rev., 1997, 97, 3425, and references cited therein. CrossRef
10. (a) E. Negishi, Organometallics in Organic Synthesis, Wiley, New York, 1980; (b) J. F. Hartwig, Organotransition Metal Chemistry, From Bonding to Catalysis, University Science Books, California, 2010.
11. Review on reactive enolates generated via transmetalation from silyl enol ethers: I. Kuwajima and E. Nakamura, Acc. Chem. Res., 1985, 18, 181, and references cited therein. CrossRef
12. Quite recently, lithium cation complexation with an alkene was reported to accelerate radical addition reaction: (a) K. Vyakaranam, J. B. Barbour, and J. Michl, J. Am. Chem. Soc., 2006, 128, 5610; CrossRef (b) K. Vyakaranam, S. Korbe, and J. Michl, J. Am. Chem. Soc., 2006, 128, 5680; CrossRef (c) A. H. C. Horn and T. Clark, J. Am. Chem. Soc., 2003, 125, 2809; CrossRef (d) T. Clark, J. Chem. Soc., Chem. Commun., 1986, 1774. CrossRef
13. The equilibrium geometries were checked by frequency calculations in which all the eigenvalues are positive, while the transition states have only one negative eigenvalue corresponding to the reaction coordinate. ωB97X-D: The long range corrected latest functional from Head-Gordon and coworkers, which includes empirical dispersion. J.-D. Chai and M. Head-Gordon, Phys. Chem. Chem. Phys., 2008, 10, 6615; CrossRef TZVP: The valence triple-zeta basis sets with polarization functions developed by Ahlrichs and coworkers. A. Schaefer, C. Huber, and R. Ahlrichs, J. Chem. Phys., 1994, 100, 5829; CrossRef NBO analysis was carried out to study the orbital interaction. A. E. Reed, L. A. Curtiss, and F. Weinhold, Chem. Rev., 1988, 88, 899; CrossRef F. Weinhold and J. Carpenter, NBO version 3.1, 1988. All the DFT calculations were carried out using Gaussian09 program on TSUBAME supercomputer at Tokyo Institute of Technology and at the Research Center for Computational Science, Okazaki: Gaussian 09, Revision C.01, M. J. Frisch, G. W. Trucks, H. B. Schlegel, G. E. Scuseria, M. A. Robb, J. R. Cheeseman, G. Scalmani, V. Barone, B. Mennucci, G. A. Petersson, H. Nakatsuji, M. Caricato, X. Li, H. P. Hratchian, A. F. Izmaylov, J. Bloino, G. Zheng, J. L. Sonnenberg, M. Hada, M. Ehara, K. Toyota, R. Fukuda, J. Hasegawa, M. Ishida, T. Nakajima, Y. Honda, O. Kitao, H. Nakai, T. Vreven, J. A. Montgomery, Jr., J. E. Peralta, F. Ogliaro, M. Bearpark, J. J. Heyd, E. Brothers, K. N. Kudin, V. N. Staroverov, R. Kobayashi, J. Normand, K. Raghavachari, A. Rendell, J. C. Burant, S. S. Iyengar, J. Tomasi, M. Cossi, N. Rega, J. M. Millam, M. Klene, J. E. Knox, J. B. Cross, V. Bakken, C. Adamo, J. Jaramillo, R. Gomperts, R. E. Stratmann, O. Yazyev, A. J. Austin, R. Cammi, C. Pomelli, J. W. Ochterski, R. L. Martin, K. Morokuma, V. G. Zakrzewski, G. A. Voth, P. Salvador, J. J. Dannenberg, S. Dapprich, A. D. Daniels, Ö. Farkas, J. B. Foresman, J. V. Ortiz, J. Cioslowski, and D. J. Fox, Gaussian, Inc., Wallingford CT, 2009.