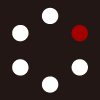
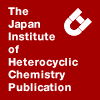
HETEROCYCLES
An International Journal for Reviews and Communications in Heterocyclic ChemistryWeb Edition ISSN: 1881-0942
Published online by The Japan Institute of Heterocyclic Chemistry
e-Journal
Full Text HTML
Received, 19th August, 2014, Accepted, 22nd September, 2014, Published online, 25th September, 2014.
DOI: 10.3987/REV-14-805
■ Synthetic Approaches to Spiro-oxindoles and Iminoindolines Based on Formation of C2–C3 Bond
Chihiro Tsukano and Yoshiji Takemoto*
Graduate School of Pharmaceutical Sciences, Kyoto University, 46-29 Yoshida-shimoadachi-machi, Sakyo-ku, Kyoto 606-8501, Japan
Abstract
Spirooxindole and iminoindoline structures can be found in a wide range of complex natural products, and compounds containing these groups invariably display interesting biological activities. Considering their interesting biological properties and complex architecture, significant research efforts have been directed towards developing new synthetic methods for constructing these structures. This review summarizes our studies towards developing novel synthetic strategies capable of accessing these structures, as well as the applications of these methods to synthesizing natural products, including elacomine and dehaloperophoramidine.CONTENTS
1. Spirooxindole
1-1. Reported Synthetic Methods for Spirooxindole by Forming C2−C3 Bond
1-2. Our Synthetic Strategies
1-3. Construction of Spirooxindole Skeleton by Domino Cyclization
1-4. Formal Synthesis of Elacomine by the Domino Palladium(0)-Catalyzed Spirooxindole Formation
1-5. Synthesis of Spirooxindoles using Silyl Amidation
2. Synthetic Studies on 2-Iminoindoline
2-1. Reported Synthetic Methods for Constructing 2-Iminoindolines and Related Compounds
2-2. SmI2-Mediated Reductive Cyclization of Carbodiimides for Synthesis of Spiro-2-Iminoindolines
2-3. Synthetic Studies of Perophoramidine
3. Summary
1. SPIROOXINDOLE
The spirooxindole structure (1) is found in many complex natural products, and several compounds containing a spirooxindole moiety have been reported to possess interesting biological properties (Figure 1).1 For example, prosurugatoxin (2),2 alstonisine (3)3 and spirotryprostatin A (4),4 which are complex pentacyclic indole alkaloids bearing contiguous stereocenters, have been reported to exhibit anti-nicotinic, moderate in vitro antiplasmodial, and antimitotic activities, respectively. The related compounds scholarisine A (5) and arbophylline (6), however, have not been reported to possess any interesting biological properties,5,6 although these molecules have captured the attention of synthetic chemists all over the world because of their unusual cage-like structures. Interestingly, various spiro-ring systems have been reported in spirooxindole natural products, such as those fused with pyrrolidine [e.g., alstonisine (3), spirotryprostatin A (4), and elacomine (7)7], piperidine [e.g., tabernoxidine (9)8], five- and six-membered carbocycles [e.g., prosurgatoxin (2) and gelsemine (10)9], and six-membered oxacycles [e.g., scholarisine A (5) and arbophylline (6)]. The spirooxindole structure is also an important pharmacophore in medicinal chemistry,10 and structures of this type can also be used as intermediates for synthesizing other indole alkaloids. Considering their numerous applications and interesting properties, there has been significant interest in developing new methods for the construction of spirooxindoles from researchers working in both synthetic and medicinal chemistry.
1-1. REPORTED SYNTHETIC METHODS FOR SPIROOXINDOLE BY FORMING C2–C3 BOND
A variety of methods have been reported for synthesizing spirooxindoles,11 and these methods can be divided into two categories, including (i) stepwise synthesis involving the formation of the oxindole ring followed by the construction of the spiro-ring system, and (ii) direct synthesis involving the simultaneous construction of the two rings making up the spiro-ring system. 3,3-Disubstituted oxindoles are quite often employed as synthetic intermediates for the stepwise synthesis of spirooxindoles because a variety of methods, including asymmetric procedures, have been developed for the synthesis of these compounds. However, the development of a direct method for the simultaneous construction of a spiro-center and an oxindole skeleton would be desirable from the perspective of synthetic efficiency. Murphy12 and Overman13 reported the development of an elegant one-step procedure for synthesizing the spiro[indoline-3,3′-pyrrolidine] skeleton using a radical-mediated or palladium-catalyzed tandem cyclization and intramolecular trapping reaction. Several other methods have also been reported for constructing spirooxindole structures in a single step, including oxidative rearrangement,14 Heck,15 intramolecular Mannich,16 ring expansion,17 and 1,3-dipolar cycloaddition18 reactions. Despite the large number of methods that have been reported in this area, research towards developing new methods for constructing spirooxindoles continues to attract considerable attention.
1-2. OUR SYNTHETIC STRATEGIES
We developed a cyanoamidation process for synthesizing 3,3-disubstituted oxindoles, which involved the formation of the C2−C3 bond (Scheme 1).19 The reaction of compound 11 with Pd(PPh3)4 in xylene at 130 °C proceeded regioselectively to give 3,3-disubstituted oxindole 12 without any of the six membered lactam.19a,b We also developed an enantioselective version of this cyanoamidation process using Pd(dba)2 with the chiral phosphoramidite 13 and N,N-dimethylpropylene urea (DMPU).19c,d It was envisaged that several new approaches could be developed for constructing the spirooxindole skeleton by extending the idea of the cyanoamidation reaction used for the formation of the C2−C3 bond, including (i) a domino reaction for the construction of the spiro-ring system; and (ii) a stepwise reaction for constructing various spiro-ring systems (Scheme 2). The first half of this review has been focused on our most recent research efforts that explore these two strategies and their application to the synthesis of natural products.20-22
1-3. CONSTRUCTION OF SPIROOXINDOLE SKELETON BY DOMINO CYCLIZATION
When we started our research towards developing of a novel domino reaction strategy for constructing spirooxindole skeletons, we had already developed a palladium catalyzed cyanoamidation process for constructing 3,3-disubstituted oxindoles from 2-(alkenyl)phenylcarbamoyl cyanide 11, as described above (Scheme 1).19 However, this cyanoamidation reaction only worked for substrates bearing a terminal olefin, which represented a major limitation for this transformation. It was envisaged that the use of a diene instead of a terminal olefin would allow 13 to react to give the π-allylpalladium intermediate 17,23 which could be converted to the spirooxindole 18 by a trapping reaction involving an intramolecular nucleophile (Scheme 3).24 With this in mind, we focused our initial efforts on the development of a palladium catalyzed cyclization reaction for carbamoyl chlorides such as 13 (X = Cl) bearing a 1,3-diene moiety.25
We initially examined an intermolecular reaction to determine the most appropriate nucleophiles for the effective trapping of the π-allylpalladium intermediate. The treatment of carbamoyl chlorides 19 bearing a diene moiety with a catalytic amount of palladium acetate (Pd(OAc)2), triphenylphosphine (PPh3) and cesium carbonate (Cs2CO3) gave the corresponding π-allylpalladium intermediates, which were trapped with several nucleophiles (Table 1). As expected, the reaction with p-NsNH2 proceeded smoothly to give the 1,4-adduct 20a via the corresponding π-allylpalladium intermediate, with none of the 1,2-adducts being observed (Table 1, entry 1). Two other sulfonamides, TsNH2 and TfNH2, were also used as nucleophiles in the reaction, resulting in the formation of oxindoles 20b and 20c (Table 1, entries 2 and 3). Although the reactions of 19 with morpholine and aniline gave the corresponding ureas 21d and 21e instead of the cyclized products 20d and 20e, the use of indole as a nitrogen nucleophile resulted in the successful formation of the cyclized product 20f (Table 1, entries 4−6). Interestingly, the π-allylpalladium intermediate also reacted with phenol and benzoic acid, which are both only weakly nucleophilic, to give the corresponding oxindoles 20g and 20h in 81% and 74% yields, respectively (Table 1, entries 7 and 8). Furthermore, the π-allylpalladium intermediate could be trapped with organoboronic acids under the same reaction conditions to give the corresponding arylated and alkenylated adducts 20i and 20j (Table 1, entries 9 and 10).
Following on from our successful synthesis of several 3,3-disubstituted oxindoles via the trapping of a π-allylpalladium intermediate with a range of nucleophiles, we proceeded to investigate the direct synthesis of spirooxindoles based on an extension of this approach. This extended approach involved sequential palladium(0)-catalyzed amidation and intramolecular nucleophilic addition reactions, which were conducted in a single pot (Scheme 4). Carbamoyl chloride 26 was prepared from aryl iodide 22 via a six-step linear sequence, including a Stille coupling with trimethylstannyl-1,3-hexadiene 23,26 as well as the introduction of a nitrogen unit using TfNH2. The domino cyclization of 26 was conducted in the presence of 10 mol% Pd(OAc)2, 20 mol% PPh3 1.2 equiv. of Cs2CO3 (1.2 equiv) at 130 °C, and gave the desired spirocyclic products 27a and 27b in a combined yield of 71% as a 63:37 mixture of two diastereomers. The newly generated stereochemistry was determined by extensive 2D NMR measurements, including NOE experiments. Notably, the major product 27a possessed the same configuration as spirotryprostatin A (4) and elacomine (7) (Figure 1).
A novel domino palladium-catalyzed method was developed by our group for the direct synthesis of spirooxindole 27 from carbamoyl chloride 26 bearing a terminal 1,3-diene moiety.20 Although the selectivity of the spiro-ring formation was poor, we proceeded to investigate the application of this palladium-catalyzed spirooxindole formation to the formal synthesis of elacomine (7) and isoelacomine (8).
1-4. FORMAL SYNTHESIS OF ELACOMINE BY THE DOMINO PALLADIUM(0)-CATALYZED SPIROOXINDOLE FORMATION
Elacomine (7, Figure 1) is a hemiterpene spirooxindole alkaloid, which was first isolated from the shrub Elaeagnus commutata in 1968 by Slywka et al.7a The structure of elacomine was determined by X-ray crystallography by James and Williams.7b Borschberg et al.27 later reported that 7 occurred naturally in its racemic form following a series of re-isolation studies. The first enantioselective total synthesis of 7 and its diastereomer 8, which is known as isoelacomine and was isolated from the same species, was described by Borschberg in the same paper, and proceeded via the oxidative rearrangement of β-carboline. The authors of this particular study also reported that elacomine and isoelacomine could be interconverted and undergo racemization under mild conditions. Although compounds 7 and 8 have not been reported to exhibit any biological activity, these molecules are regarded by many researchers as important synthetic targets and benchmark compounds, because they contain a simple spiro(pyrrolidine-3,3-oxindole) skeleton that is often found in spirooxindole natural products. In 2004, Horne et al.28 reported the total synthesis of 7 and 8 based on a stereoselective intramolecular iminium ion spirocyclization method. White et al.29 developed a tandem intramolecular photocycloaddition retro-Mannich fragmentation strategy and applied it to the diastereoselective synthesis of 7. Notably, tryptamine derivatives were selected as starting materials in all three of these total syntheses, with the main focus of each approach being the construction of the spiro-center.
We envisioned that the application of our newly developed domino reaction to an internal 1,3-diene would allow for the efficient construction of the spirooxindole skeleton of elacomine (7) and isoelacomine (8) from carbamoyl chloride 29, which would act as a cyclization precursor (Scheme 5). Oxidative addition and insertion into the 1,3-diene would give the π-allylpalladium complexes 30a and 30b as well as the oxindole. Intramolecular nucleophilic attack to the π-allylpalladium moiety would give compound 28. Considering the acidity required to generate a suitably nucleophilic nitrogen-based nucleophile and the feasibility of the subsequent deprotection, a methoxy carbamate was chosen as an internal nitrogen-based nucleophile (i.e., R2 = Me). Carbamoyl chloride 29 could be synthesized in several steps, including the Heck reaction of iodoaniline 31 and dihydro-2H-pyranone 32 followed by the ring-opening of the dihydro-2H-pyranone moiety to allow for the introduction of the nitrogen and isoprenyl units, and the formation of the carbamoyl chloride.
Practical work towards realizing this strategy was initially focused on the intermolecular Heck reaction of dihydro-2H-pyranone 32, because the reaction of this species had not been reported in the same way as the intermolecular Heck reaction of cyclic enones.30 Screening experiments revealed that 31a could be coupled to 32 using Pd(OAc)2, PPh3, iPr2NEt and tetrabutylammonium bromide (Bu4NBr) in dimethylformamide (DMF) at 100 °C followed by deprotection of the t-butoxycarbonyl (Boc) group to give lactone 33 in a 52% yield over the two steps (Scheme 6). The diene unit was introduced by the half-reduction of lactone 33 using diisobutylaluminum hydride (DIBAL-H) followed by a Wittig reaction. Trifluoroacetylation of the aniline moiety followed by mesylation of the primary alcohol gave 35, which was treated with NaN3 to give the corresponding azide 36. Reduction of the azide followed by the protection of the resulting amine as a methoxy carbamate gave compound 37, which was treated with KOH to allow for the deprotection of the trifluoroacetyl group. The resulting secondary amine 38 was treated with a mixture of triphosgene and pyridine in CH2Cl2 at –78 °C to give carbamoyl chloride 29a, which could be used as a cyclization precursor.
We subsequently investigated the domino palladium-catalyzed spirooxindole formation of carbamoyl chloride 29a bearing an internal diene moiety. Compound 29a was initially subjected to a set of conditions that had been optimized for terminal dienes, such as those shown in Scheme 4.20 Unfortunately, however, the treatment of 29a with Pd(OAc)2, PPh3 and Cs2CO3 in xylene at 130 °C gave compound 39 in a 86% yield, which was most likely produced by the β-elimination of the π-allylpalladium complex instead of the nucleophilic addition of the nitrogen atom (Table 2, entry 1). Several additives and ligands were screened in an attempt to suppress the undesired β-elimination. Disappointingly, the use of diphenylphosphinoferrocene (dppf) as a ligand or Bu4NPF6 as an additive in the presence of Cs2CO3 or Ag2CO3 proved to be unsuccessful, whereas the addition of Bu4NI led to the formation of 28 in only 11% yield with no diastereoselectivity (Table 2, entries 2−5). Interestingly, the reaction proceeded smoothly to give the desired spirooxindole 28 as the major product when it was conducted without a base such as Cs2CO3 (Table 2, entry 6). Given that the undesired β-elimination step could potentially be suppressed under acidic conditions, we investigated the addition of several acids to the reaction mixture, including TfOH, Hf(OTf)4 and Bi(OTf)3 (Table 2, entries 7−9). The results of these experiments revealed that Bi(OTf)3 was an effective additive for this domino palladium-catalyzed spirooxindole formation (Table 2, entry 9). The resulting spirooxindole 28 was isolated as a 1:1 mixture of diastereomers in all cases because the inversion of the π-allylpalladium intermediates 30a and 30b would occur prior to the nucleophilic addition of the nitrogen atom (Scheme 5).
The Lewis acid catalyzed intramolecular hydroamination of 39 was investigated to determine whether diene 39 was formed as an intermediate in the domino cyclization reaction described above. The treatment of 39 with a Bi(OTf)3 or Hf(OTf)4 catalyst in xylene at 130 °C according to the conditions described above for the domino cyclization reaction, except for the absence of the palladium catalyst and ligand, gave spirooxindole 28 as a mixture of diastereomers in 49 and 41% yields, respectively (Table 3, entries 1 and 2). The use of Shibasaki’s conditions,31 which involved the use of a PF6 salt and Bi(OTf)3, Hf(OTf)4 or Sc(OTf)3, led significant improvements in the yield, with a combination of Bi(OTf)3 and KPF6 being especially effective (Table 3, entries 3−6).32 In contrast, the use of Zn(OTf)2 did not afford any of the cyclized products (Table 3, entry 7). The diastereoselectivity of the cyclization was found to be 1:3 under almost all of the conditions tested, and therefore different to the results observed in the domino cyclization process. These results therefore indicated that 39 was not an intermediate in the domino cyclization reaction. A comparison of these results with those from the hydroamination reaction suggested that nucleophilic addition to the π-allylpalladium complex would be preferred to the hydroamination pathway in the domino reaction.
Having successfully synthesized spirooxindole 28, which possessed the same carbon skeleton as elacomine (7) and isoelacomine (8), we turned our attention to the final stage of the synthesis. The hydrogenation of 28 under high pressure conditions gave diastereomers 40a and 40b, which were separated by column chromatography over silica gel (Scheme 7). Finally, the formal synthesis of elacomine and isoelacomine was achieved by the removal of the benzyl group from the nitrogen atom of the isoxazole ring with lithium di-t-butylbiphenyl (LiDBB). The spectral data for the key intermediates 41a and 41b were in agreement with those reported by Horne et al.28
This newly developed domino palladium-catalyzed spirooxindole formation was successfully applied to the formal synthesis of elacomine (7) and isoelacomine (8). It is noteworthy that terminal and internal dienes could both be employed in this domino cyclization.21
1-5. SYNTHESIS OF SPIROOXINDOLES USING SILYL AMIDATION
The focus of our research subsequently shifted to synthesizing spirooxindoles fused with tetrahydropyran, piperidine, and five-membered carbocycle ring systems. Developing a unified synthetic strategy capable of providing access to various spirooxindoles would be of considerable value to synthetic and medicinal chemists because it could be applied to the synthesis of natural products as well as compound libraries for use in drug discovery. The domino cyclization reaction described above20,21 is a convenient method for constructing spiro-ring systems fused with pyrrolidine, although the scope of this reaction has been limited by its requirement for strong nucleophiles to allow for the intramolecular trapping of the π-allylpalladium intermediate. For this reason, the domino cyclization reaction could be unsuitable for the synthesis of other spiro-ring systems. To develop a comprehensive method capable of providing access to various spirooxindole systems, we turned our attention to the bismetallation of 1,3-dienes, which provides products containing two carbon–metal bonds that could be subjected to further transformations.33 Mori et al.34 developed a nickel-catalyzed process for the bismetallative cyclization of 1,3-dienes with an aldehyde on their side chain. Furthermore, the same reaction with Me3SiSnBu3 gave a cyclized product containing an allylstannyl group. This reaction was extended to include the formation of two carbon–carbon bonds by sequential allylic transfer reactions in one pot by Yu.35 The nickel-catalyzed cyclization of 1,3-dienes with internal and external aldehydes in the presence of a diboronyl reagent proceeded in high levels of diastereoselectivity. Based on this bismetallative cyclization process, we became interested in developing a carbosilylation strategy for constructing various spirooxindole ring systems. We envisioned that the treatment of 1,3-diene 13 bearing a carbamoyl chloride with a catalytic amount of palladium catalyst and hexamethyldisilane would give the disubstituted oxindole 42 via the reaction of the π-allyl complex 41 with the internal carbamoyl chloride (Scheme 8). The C1 position of the diene unit would be converted to a quaternary carbon center and the resulting oxindole 42, bearing an allylic silanyl group, could be readily converted to spirooxindole 43 by the Sakurai-type reaction of the tethered electrophile (R"), which would allow for a high level of control over the three contiguous stereocenters. Although the realization of this strategy would mean that the spirooxindole ring would have to be formed in a stepwise manner, various spiro-ring systems could be accessed from this intermediate by the nucleophilic attack of the allyl metal species on the electrophile in the side chain. Conceptually, this idea is effectively the reverse of that described above for the domino cyclization reaction in terms of the nucleo- and electro-philicity of the allyl metal intermediate derived from the diene.
When we started our work in this area, there had been no reports in the literature pertaining to the palladium-catalyzed bismetallative cyclization of a carbamoyl chloride with a 1,1-disubstituted diene. In the absence of any related examples to guide our research, our initial efforts focused on the carbosilylation of 1,3-diene 19 using hexamethyldisilane and 10 mol% of a palladium or rhodium catalyst in xylene (Table 4). Although the use of Rh(PPh3)3Cl as a catalyst resulted in none of the desired cyclization product, the use of the chloro(1,5-cyclooctadiene)rhodium(I) dimer ([Rh(cod)Cl]2) gave 44 in 43% yield as a mixture of (E)- and (Z)-isomers (Table 4, entries 1 and 2).36 Several palladium catalysts were screened against this reaction and performed effectively in the cyclization to give the desired product 44 in 22%–75% yields (Table 4, entries 3–7).37 Interestingly, however, the addition of PPh3 hampered the cyclization reaction (Table 4, entries 8 and 9). Finally, we found that the allylpalladium(II) chloride dimer [Pd(η3-allyl)Cl]2 was an efficient catalyst for this transformation, with 5 mol% catalyst allowing the reaction to proceed to completion within 1 h to afford the desired product 44 in 77% yield (Table 4, entry 10).
The optimal conditions were subsequently applied to a variety of substrates to assess the scope of this reaction (Table 5). Carbamoyl chlorides 45a–c were prepared from Boc-protected 2-iodoaniline, because the presence of a siloxyethyl or aminoethyl group was essential for the formation of the spiro-ring. Compounds 45a–c were subsequently converted to the desired oxindoles 46a–c in 75%−95% yields using 5 mol% [Pd(η3-allyl)Cl]2 and hexamethyldisilane. The substrate scope of this reaction was also investigated using carbamoyl chlorides without a siloxyethyl or aminoethyl side chain (i.e., 45d–f). A p-methoxybenzyl (PMB) group was tolerated under the cyclization conditions, with the PMB substituted product 46d being isolated in good yield. Substrates bearing a chlorine or methoxy group on their aromatic ring also reacted smoothly to give oxindoles 46e and 46f in 86% and 80% yields, respectively.
Spirooxindoles fused with a five-membered carbocycle were synthesized by a Sakurai-type reaction38 using the 3,3-disubstituted oxindole described above (Scheme 9). The removal of the t-butyldimethylsilyl (TBS) group from 46a under acidic conditions gave alcohol 47, which was converted to enol ether 48 following sequential oxidation and Wittig reactions. Enol ether 48, which existed as a 2:1 mixture of E:Z isomers, was converted to spirooxindole 49 under acidic conditions in 89% yield as a 5:3 mixture of diastereomers. The formation of spirooxindoles fused with a six-membered piperidine ring was also achieved with excellent diastereoselectivity. The Mitsunobu reactions of compound 47 with succinimide and glutarimide gave the corresponding addition products, which were reduced with NaBH4 to give the cyclization precursors 50a and 50b, respectively. Treatment of compounds 50a and 50b with BF3·OEt2 gave compounds 51a and 51b in 95% and 98% yields, respectively, with excellent diastereoselectivity, and the newly generated stereochemistry was confirmed by 2D NMR analyses, including NOESY experiments. Notably, these products possessed the same relative configuration as the core structure of tabernoxidine (9) (Figure 1).8
The 3,3-disubstitued oxindole 46c was used to synthesize a series of spirooxindoles fused with a tetrahydropyran ring using a Sakurai-type cyclization. Deprotection of the TBS group in compound 46c gave the corresponding alcohol 52, which was treated with trimethyl orthoformate or an aryl aldehyde in the presence of BF3·OEt2 to give the spirooxindoles 53a–e in high yields (Table 6). The reaction with trimethyl orthoformate gave 53a in 83% yield as a single diastereomer, and several other aldehydes, including electron-rich or electron-deficient aromatic aldehydes, reacted smoothly to give the desired products 53b–e as single isomers in 78%–89% yields.
The excellent diastereoselectivity observed in this cyclization can be rationally explained as follows: It would be possible for the 3,3-disubstitued oxindole substrates to adopt four chair-like transition states A–D via the formation of an oxonium cation (Figure 2). Given that an aromatic ring is more sterically encumbered than an amide group,39 transition state A, where the aromatic ring and allylic silane group are both sitting in pseudo-equatorial positions, would be energetically more favorable than transition states B–D.
The examples shown above provide a good illustration of the powerful and concise nature of this newly developed method for constructing various spirooxindoles via the palladium-catalyzed carbosilylation of 1,3-dienes bearing a carbamoyl chloride moiety followed by a Sakurai-type cyclization.22 Spiro-ring systems fused with tetrahydropyran, piperidine, and five-membered carbocycles can be constructed according to this method with a high level of control over two or three contiguous stereocenters. Furthermore, the work described here was the first reported example of the construction of a quaternary stereocenter from the 1,1-disubstituted diene via a bismetallation strategy.40
Using this methodology, it is now possible to access spirooxindole systems fused with pyrrolidine, piperidine, five-membered carbocycle and six-membered lactone rings based on the originally developed method. These two synthetic strategies could therefore not only be useful for synthesizing natural spirooxindoles but could also be used to synthesize the intermediates of indole alkaloids.
2. SYNTHETIC STUDIES ON 2-IMINOINDOLINE
During the course of our synthetic studies of spirooxindoles, we became interested in synthesizing 2-iminoindolines (54a), 2-aminoindolenines (54b) and 2-aminoindolines (55) (Figure 3). In some cases, 54a and 54b could be tautomers of each other. The class of natural products containing these structures is very similar to that of spirooxindoles, and several interesting examples from this class have been reported in the literature, including communesin F (56),41 perophoramidine (57),42 flustramine C (58),43 quinadoline B (59)44 and neoxaline (60)45 (Figure 4). These compounds often exhibit interesting biological activities, and communesin F (56), perophoramidine (57) and neoxaline (60), for example, have been reported to be cytotoxic. Flustramine C (58) shows moderate antibiofilm activity against Acinetobacter baumannii, Escherichia coli and methicillin resistant Staphylococcus aureus (MRSA);46 and quinadoline B (59) has been reported as a moderate inhibitor of lipid droplet synthesis in mouse macrophages. The synthesis of these compounds, however, is challenging because of their complex structural architecture. In the latter half of this review, we will focus in detail on our synthetic efforts towards developing a robust strategy for constructing 2-iminoindolines.47,48
2-1. REPORTED SYNTHETIC METHODS FOR CONSTRUCTING 2-IMINOINDOLINES AND RELATED COMPOUNDS
Various methods have been reported in the literature for synthesizing 2-iminoindolines and related compounds. Most of the reported methods involve the tether-dependent condensation of oxindole derivatives49a–d and the oxidative cyclization of indoles.49e Several other synthetic strategies have also been developed to provide access to these systems, including aza-Pauson–Khand-type reactions,50 heteroannulation of N-alkynylanilines,51 ring enlargement of aminoazirines,52 addition of azides to indoles,53 oxidation of aminals,54 and amidination of aryl halides.55 Despite several methods being available for constructing these compounds, the development of mild and selective synthetic methods is still highly desired.
2-2. SMI2-MEDIATED REDUCTIVE CYCLIZATION OF CARBODIIMIDES FOR SYNTHESIS OF SPIRO-2-IMINOINDOLINES
For the synthesis of spiro-2-iminoindolines 61, we envisioned a retrosynthetic disconnection of the C2−C3 bond because we had already developed a synthetic method for constructing spirooxindoles by forming the C2−C3 bond (Scheme 10). Compound 62 bearing an unsaturated carbonyl group and a carbodiimide moiety was therefore identified as a suitable synthetic precursor for the cyclization reaction. Carbodiimides are often employed as synthetic precursors for amidines for their reactions with various organometallic species.56 However reports pertaining to their use as radical acceptors have been scarce.57
SmI2, which was first introduced as a reagent for synthetic chemistry in 1977 by Kagan, is a powerful single-electron reductant.58,59 SmI2 exhibits excellent functional group selectivity and is regularly used to affect the formation of carbon–carbon bonds and selective reduction reactions in synthesizing complex natural products.60 Although various reductive homo-/hetero-coupling reactions have been reported involving the reaction of SmI2 with substrates bearing electron-withdrawing groups,61 there have, to the best of our knowledge, been no reports to date pertaining to the development of similar reactions involving carbodiimides.62
Before we started this project, a report appeared in the literature describing the synthesis of oxindoles based on the SmI2-promoted intramolecular cyclization of aryl isocyanates bearing a cyclohexenone moiety as part of a synthetic study towards welwitindolinone A isonitrile.61g Inspired by these results, it was envisioned that a quaternary-carbon center and an imine carbon bond could be constructed via a reductive cyclization reaction involving SmI2, an α,β-unsaturated carbonyl moiety and carbodiimide. According to this strategy, the radical or anionic species generated from the reaction of SmI2 with an unsaturated carbonyl group would attack the central carbon atom of the carbodiimide species, or vice versa. Although tandem nucleophilic addition and 6π electrocyclic reactions have been reported for the related carbodiimides 62, which gave the corresponding dihydroquinazolines 63 and 2-aminoquinoline 64, respectively,63,64 there have been no reports concerning similar cyclization reactions for 62 to give quaternary-carbon centers (Scheme 11).
Compound 65 was selected as a simple model substrate to establish suitable conditions for the reductive cyclization reaction. Treatment of THF solution of 65 with SmI2 at −78 °C resulted in no reaction (Table 7, entry 1), although the desired reaction did proceed at room temperature to give the cyclized product 66 exclusively (Table 7, entry 2). The addition of an additive to the reaction was also investigated, with hexamethylphosphoramide (HMPA)65 and t-butanol (tBuOH) providing similar enhancements in the yield of the cyclized product (Table 7, entries 3 and 4). In contrast, the addition of several other additives, including LiCl66a and NiI266b did not lead to any significant improvements in the yield (Table 7, entries 5 and 6). The structure of iminoindoline 66 was unambiguously determined by the X-ray crystallographic analysis of 67, which was prepared by the Boc protection of 66 (Figure 4).
The scope and limitations of this SmI2-mediated reductive cyclization reaction were evaluated using a series of carbodiimides, and the results are shown in Table 8. Some of the cyclized products were found to be unstable, and the cyclization yields were therefore determined following the regioselective Boc protection of amidine. Under the optimal reaction conditions (method A), both electron-donating and electron-withdrawing functionalities were well tolerated on the phenyl ring to the left-hand side of the carbodiimide, including chlorine, methyl and methoxy groups, which gave the spirocyclic products 69a–d in 62% to 87% yields. It is noteworthy that compound 68a, bearing a conformationally restricting substituent at the 3-position of its aromatic ring, reacted smoothly to give the desired product in good yield. When substrates 68e−i bearing substituents on the phenyl ring to the right-hand side of the carbodiimides were used in the reaction, the optimized conditions (method A) gave lower yields of the cyclized products as well as several unidentified byproducts. Further experimental work concerning substrates 68e−i revealed that the use of an inverse addition and changes to the amounts of SmI2 and t-butanol to the reaction (Table 8, condition B) led to much higher yields with fewer byproducts. In the case of 68e, a small amount of byproduct was formed from a 6π electrocyclic reaction, which most likely occurred as a result of Lewis acid-promoted acceleration through a chelated Sm species.
When the acyclic unsaturated ester 68j and lactone 68k were subjected to the optimal reaction conditions (condition B) they gave the desired products 69j and 69k in 76% and 83% yields, respectively (Table 8). In sharp contrast, the reaction of the corresponding ethyl cinnamate 68l resulted in a complex mixture. Analysis of this mixture by mass spectroscopy revealed the presence of dimerized and trimerized products. These results therefore indicated that the β,β-disubstituted carbonyl moiety was essential to the success of the reaction.
A possible mechanism for this reductive cyclization reaction is shown in Scheme 12. The carbonyl oxygen of compound 68 would initially coordinate to SmI2. The subsequent reduction of the unsaturated carbonyl group would produce a radical anion 70 that would attack the carbon of the carbodiimide at a faster rate than the samarium enolate. Further reduction by a second equiv. of SmI2 would provide the Sm amidinate 71. Given that cyclized product was formed in the absence of t-butanol, dianion 71 would be generated without the assistance of a proton source (Table 7, entry 2). Finally, protonation of 71 would give the reductively cyclized iminoindoline 69. However, it remains unclear whether the formation of the carbon–carbon bond is promoted by chelation between a carbodiimide moiety and SmI2 prior to the cyclization. The reported instability and difficulties associated with the generation of nitrogen-centered radical species57 suggest that dianion 71 could be formed from a chelated complex.
The SmI2-promoted intramolecular reductive cyclization reaction of carbodiimides bearing unsaturated carbonyl moieties was developed for the synthesis 2-iminoindolines bearing quaternary-carbon centers at their C3 position.47 We considered this newly developed synthetic method is a powerful tool for the construction of 2-iminoindolines, and consequently applied this strategy to synthesize the core structure of perophoramidine (57).
2-3. SYNTHETIC STUDIES OF PEROPHORAMIDINE
Perophoramidine (57) was isolated from an extract of Philippine ascidian Perophora namei by Ireland et al.42 in 2002 (Figure 3). The structure of this compound is characterized by a pentacyclic alkaloid bearing two contiguous quaternary carbon stereocenters, and was determined following extensive spectroscopic analyses, including a series of 2D NMR experiments. Perophoramidine (57) has been reported to induce apoptosis via PARP cleavage as well as being cytotoxic towards HCT116 colon carcinoma cells, with an IC50 of 60 μM. Significant research efforts have been directed towards the synthesis of this natural product because of its highly complex polycyclic structure and interesting biological activities.67-75 In 2004, Funk et al.67a accomplished the first total synthesis of (±)-perophoramidine, and its absolute configuration was confirmed by Qin in 2010 following his total synthesis of (+)-perophoramidine.68a The key step in all of these syntheses was the Diels–Alder reaction of an indole with an ortho-quinone methide imine, which was inspired by the proposed biosynthetic pathway.69a Rainier also described a synthesis of (±)-dehaloperophoramidine 72,71 and Wang recently reported the total synthesis of (+)-perophoramidine, which involved the catalytic asymmetric alkylation reaction of a 3-bromooxindole as a key step.75 We also became interested in the total synthesis of perophoramidine because we had developed a synthetic method for constructing 2-iminoindolines.
Dehaloperophoramidine 72 was selected as our initial target, because this compound can be synthesized from the pentacyclic compound 73 according to an existing procedure (Scheme 13).71 The key features of our retrosynthesis can be summarized as follows: (i) palladium-catalyzed intramolecular C−N bond formation between the amidine nitrogen and the aryl chloride in spiro-2-iminoindoline 74; and (ii) the SmI2-mediated reductive cyclization of carbodiimide 75 bearing an electron-deficient tetrasubstituted olefin. This strategy was reliant on the aryl chloride moiety of 75 remaining intact throughout the SmI2-mediated reductive cyclization reaction so that it could then be used in the palladium-catalyzed cyclization. Our strategy for this compound was unique in terms of the way in which we planned to assemble the 3,3-disubstituted 2-iminoindoline during the initial stage of the process. In contrast, Funk, Qin, Rainier, and Wang had all employed indoles and their oxidized intermediates during the latter stages of their respective processes for constructing the core structure.
Carbodiimide 75, which contains a tetrasubstituted olefin, was synthesized from the commercially available ethyl N-(benzylamino)propionate (76) (Scheme 14). The introduction of the ethyl malonyl moiety followed by the Dieckmann condensation of the resulting amide gave lactam 77, which was converted to β-keto-lactam 78 via a one-pot hydrolysis decarboxylation reaction. Treatment of 78 with N-phenyl trifluoromethanesulfonimide (Tf2NPh) and Et3N gave the corresponding vinyl triflate 79. The Suzuki coupling reaction of triflate 79 with boronic acid 8076 gave the biaryl product 81, which was sequentially brominated and trityl (Tr)-protected to give 82. The second Suzuki coupling of lactam 82 with 2-chlorophenyl boronic acid 83 proceeded smoothly to give the tetrasubstituted olefin, which was treated with trifluoroacetic acid to give aniline 84 in 50% yield from 82. Two carbodiimides (75a and 75b) bearing a p-methoxyphenyl (PMP) or p-methoxybenzyl (PMB) group were synthesized to determine the effect of different protecting groups on the palladium-catalyzed intramolecular C–N bond forming reaction. These carbodiimides were prepared by the treatment of compound 84 with an appropriate aryl isocyanate followed by the dehydration of the resulting urea with triphenylphosphine and carbon tetrabromide. The resulting carbodiimides 75a and 75b were found to be stable at room temperature, whereas carbodiimide 65, which did not possess an α-substituent on its α,β-unsaturated lactam, was gradually converted to 2-aminoquinoline through an undesired 6π electrocyclic reaction under the same conditions (Scheme 11 and Table 7).64
With carbodiimides 75a and 75b bearing tetrasubstituted olefins in hand, we proceeded to investigate their performance in the SmI2-mediated reductive cyclization. The reductive cyclization of the N-PMP carbodiimide 75a with SmI2 in the presence of t-butanol at room temperature proceeded smoothly to give spiro-2-iminoindoline 74a in 86% yield (Scheme 14 and Table 9, entry 1). 1H NMR and X-ray crystallography indicated that 74a existed as a mixture of amidine-tautomers, with the ratio of the tautomers depending on the solvent. X-Ray crystallographic analysis of 74a also revealed that the two aryl groups on the 2-piperidinone ring were in a syn configuration (Figure 5, left). The stereoselectivity of this reaction was attributed to the selective protonation of the samarium enolate 85 from the same side as the PMP-imine moiety, which was less sterically hindered (Figure 5, right). We also investigated the reductive cyclization of N-PMB carbodiimide 75b under the optimal conditions. Unfortunately, the reaction did not proceed at room temperature or 60 °C, with the starting material 75b being recovered in both cases (Table 9, entries 2 and 3). Pleasingly, the use of HMPA as a co-solvent, which is generally used to increase the reduction potential of SmI2,65 allowed for the desired cyclization reaction to proceed at room temperature to give iminoindoline 74b in 90% yield (Table 9, entry 4).
The epimerization of the α-position of the lactam was investigated prior to evaluating the intramolecular palladium-catalyzed aryl amidination77 because the spiro-2-iminoindolines 74a and 74b were much less likely than the other diastereomers to undergo the cyclization based on their conformation. Treatment of spiro-2-iminoindoline 74a with sodium t-butoxide in DMA at room temperature resulted in no reaction, whereas 74c was obtained by epimerization after 2 h at 60 °C, which was observed by 1H NMR analysis (Scheme 15). Surprisingly, the desired cyclization reaction occurred to give the pentacyclic compound 73a when the material was heated at 120 °C for 24 h (Table 10, entry 1). While we did not extensively investigate these transition-metal-free conditions, we suspected a involvement of a trace amount of palladium catalyst under these conditions. Several alkylphosphine ligands were screened to develop a concise cyclization reaction for the palladium catalyzed amidination of compound 74a. Although the use of Ad2PBu in the presence of Pd(OAc)2 did not give satisfactory results (Table 10, entry 2), tBu3P·HBF4, CyJohnPhos, DavePhos, and tricyclohexylphosphine (Cy3P) led to improvements in the yield up to 78% (Table 10, entries 3−6). Notably, Cy3P, which was identified as the best ligand for the reaction, also led to a significant increase in the reaction rate (17 h, 78% yield) (Table 10, entry 6). Decreasing the amounts of palladium catalyst and Cy3P led to an increase in the yield up to 86% (Table 10, entry 7). The newly generated stereochemistry in pentacycle 73a was determined by 2D NMR analyses, including NOESY experiments (Figure 6). In sharp contrast, the application of these optimized conditions to the N-PMB-protected iminoindoline 74b did not give the cyclized product. In this particular case, the starting material was recovered unchanged, and the differences in the reactivities of the two substrates were attributed to differences in the electronic properties. However, it is important to mention that a detailed mechanism for this cyclization reaction has not yet been determined.
The pentacyclic skeleton of perophoramidine (57) was successfully constructed by the SmI2-mediated reductive cyclization of carbodiimide 75a bearing a tetrasubstituted olefin followed by a palladium-catalyzed intramolecular C−N bond formation.48 The resulting pentacyclic compound 73a was synthetically equivalent to Rainier’s pentacyclic amidine, which was used as an intermediate for dehaloperophoramidine.71 This newly developed and unique strategy provided a novel platform for synthesizing natural products containing an amidine moiety.
3. SUMMARY
We have been heavily involved in developing novel synthetic strategies for constructing spirooxindoles based on the formation of the C2−C3 bond. Our work in this area has led to the development of a palladium-catalyzed domino reaction for the formation of spirooxindoles, as well as an alternative strategy involving the palladium-catalyzed carbosilylation of carbamoyl chlorides bearing a 1,3-diene moiety. Using the former method, we successfully completed a formal synthesis of elacomine and isoelacomine. The combination of our newly developed domino palladium-catalyzed carbosilylation of 1,3-dienes with a Sakurai-type cyclization reaction allowed for the successful construction of spirooxindoles fused with tetrahydropyran, piperidine, and five-membered carbocycles, with control over two or three contiguous stereocenters.
Inspired by these newly developed methods for constructing spirooxindoles, we also established a SmI2-mediated process involving the intramolecular reductive cyclization of carbodiimides, which allowed for the synthesis of 2-iminoindolines bearing a quaternary-carbon center at their C3 position. This unique synthetic method was combined with a palladium-catalyzed intramolecular C−N bond formation reaction to allow for the effective construction of the core structure of perophoramidine, which is a hexacyclic alkaloid.
We believe that these synthetic strategies represent a powerful addition to the plethora of synthetic transformations already available to synthetic chemists, and could be readily applied to the synthesis of complex spirooxindole- and iminoindoline-containing natural products. Further work towards the application of these reactions is currently underway in our laboratory and will be reported in due course.78
ACKNOWLEDGEMENTS
The studies described in this review were performed by Dr. Y. Yasui, Dr. H. Kamisaki, Dr. S. M. Hande, Dr. T. Ishida, Mr. T. Nanjo and Mr. M. Nakajima, and we are deeply indebted to them for all of their hard work. These studies were supported in part by Grants-in-Aid for Scientific Research (B) (Y.T.), Young Scientists (B) (C.T.), and Young Scientists (Start-up) (Y.Y.), “Targeted Proteins Research Program” and “Platform for Drug Design, Discovery and Development” from the Ministry of Education, Culture, Sports, Science and Technology of Japan, and JSPS Research Fellowship for Young Scientists (T.I.).
References
1. J. S. Bindra, ‘The Alkaloids’, Vol. 14, ed. by R. H. F. Manske, Academic Press, Inc., New York, 1973, pp.84-121; 2014/10/03, ; CrossRef A. J. Kochanowska-Karamyan and M. T. Hamann, Chem. Rev., 2010, 110, 4489. CrossRef
2. T. Kosuge, K. Tsuji, K. Hirai, T. Fukuyama, H. Nukaya, and H. Ishida, Chem. Pharm. Bull., 1985, 33, 2890. CrossRef
3. R. C. Elderfield and R. E. Gilman, Phytochemistry, 1972, 11, 339; CrossRef S. Cheenpracha, T. Ritthiwigrom, and S. Laphookhieo, J. Nat. Prod., 2013, 76, 723. CrossRef
4. C.-B. Cui, H. Kakeya, and H. Osada, Tetrahedron, 1996, 52, 12651. CrossRef
5. X.-H. Cai, Q.-G. Tan, Y.-P. Liu, T. Feng, Z.-Z. Du, W.-Q. Li, and X.-D. Luo, Org. Lett., 2008, 10, 577. CrossRef
6. K.-H. Lim and T.-S. Kam, Tetrahedron Lett., 2006, 47, 8653. CrossRef
7. G. W. A. Slywka, Ph. D. Thesis, The University of Alberta, Edmonton; CrossRef M. N. G. James and G. J. B. Williams, Can. J. Chem., 1972, 50, 2407. CrossRef
8. B. S. Joshi, P. G. Rao, D. Rogers, B. P. Singri, and D. J. Williams, Indian J. Chem. Sect. B, 1984, 23B, 101.
9. T. G. Wormley, Am. J. Pharm., 1870, 42, 1; F. M. Lovell, R. Pepinsky and A. J. C. Wilson, Tetrahedron Lett., 1959, 1, 1; CrossRef H. Conroy and J. K. Chakrabarti, Tetrahedron Lett., 1959, 1, 6. CrossRef
10. A. Fensome, W. R. Adams, A. L. Adams, T. J. Berrodin, J. Cohen, C. Huselton, A. Illenberger, J. C. Kern, V. A. Hudak, M. A. Marella, E. G. Melenski, C. C. McComas, C. A. Mugford, O. D. Slayden, M. Yudt, Z. Zhang, P. Zhang, Y. Zhu, R. C. Winneker, and J. E. Wrobel, J. Med. Chem., 2008, 51, 1861; CrossRef N. Teno, K. Masuya, T. Ehara, T. Kosaka, T. Miyake, O. Irie, Y. Hitomi, N. Matsuura, I. Umemura, G. Iwasaki, H. Fukaya, K. Toriyama, N. Uchiyama, K. Nonomura, I. Sugiyama, and M. Kometani, J. Med. Chem., 2008, 51, 5459. CrossRef
11. A. B. Dounay and L. E. Overman, Chem. Rev., 2003, 103, 2945; CrossRef H. Lin and S. J. Danishefsky, Angew. Chem. Int. Ed., 2003, 42, 36; CrossRef C. Marti and E. M. Carreira, Eur. J. Org. Chem., 2003, 2209; CrossRef A. Steven and L. E. Overman, Angew. Chem. Int. Ed., 2007, 46, 5488; C. V. Galliford and K. A. Scheidt, Angew. Chem. Int. Ed., 2007, 46, 8748; CrossRef B. M. Trost and M. K. Brennan, Synthesis, 2009, 3003; CrossRef F. Zhou, Y.-L. Liu, and J. Zhou, Adv. Synth. Catal., 2010, 352, 1381; CrossRef R. Dalpozzo, G. Bartoli, and G. Bencivenni, Chem. Soc. Rev., 2012, 41, 7247; CrossRef N. R. Ball-Jones, J. J. Badillo, and A. K. Franz, Org. Biomol. Chem., 2012, 10, 5165. CrossRef
12. D. Lizos, R. Tripoli, and J. A. Murphy, Chem. Commun., 2001, 2732. CrossRef
13. L. E. Overman and M. D. Rosen, Angew. Chem. Int. Ed., 2000, 39, 4596; CrossRef L. E. Overman and M. D. Rosen, Tetrahedron, 2010, 66, 6514; CrossRef Y. Yasui, H. Kamisaki, T. Ishida, and Y. Takemoto, Tetrahedron, 2010, 66, 1980. CrossRef
14. S. D. Edmondson and S. J. Danishefsky, Angew. Chem. Int. Ed., 1998, 37, 1138. CrossRef
15. W. G. B. van Henugouwen, R. M. Fieseler, F. P. J. T. Rutjes, and H. Hiemstra, Angew. Chem. Int. Ed., 1999, 38, 2214; CrossRef W. G. B. van Henugouwen, R. M. Fieseler, F. P. J. T. Rutjes, and H. Hiemstra, J. Org. Chem., 2000, 65, 8317. CrossRef
16. F. Von Nussbaum and S. J. Danishefsky, Angew. Chem. Int. Ed., 2000, 39, 2178. CrossRef
17. P. B. Alper, C. Meyers, A. Lerchner, D. R. Siegel, and E. M. Carreira, Angew. Chem. Int. Ed., 1999, 38, 3186. CrossRef
18. P. R. Sebahar and R. M. Williams, J. Am. Chem. Soc., 2000, 122, 5666. CrossRef
19. Y. Kobayashi, H. Kamisaki, R. Yanada, and Y. Takemoto, Org. Lett., 2006, 8, 2711; CrossRef Y. Kobayashi, H. Kamisaki, H. Takeda, Y. Yasui, R. Yanada, and Y. Takemoto, Tetrahedron, 2007, 63, 2978; CrossRef Y. Yasui, H. Kamisaki, and Y. Takemoto, Org. Lett., 2008, 10, 3303. CrossRef
20. H. Kamisaki, Y. Yasui, and Y. Takemoto, Tetrahedron. Lett., 2009, 50, 2589. CrossRef
21. H. Kamisaki, T. Nanjo, C. Tsukano, and Y. Takemoto, Chem. Eur. J., 2011, 17, 626. CrossRef
22. S. M. Hande, M. Nakajima, H. Kamisaki, C. Tsukano, and Y. Takemoto, Org. Lett., 2011, 13, 1828. CrossRef
23. R. F. Heck, J. Am. Chem. Soc., 1963, 85, 3381; CrossRef S. D. Robinson and B. L. Shaw, J. Chem. Soc., 1963, 4806; CrossRef R. M. Rowe and D. A. White, J. Chem. Soc., 1967, 1451. CrossRef
24. B. A. Patel, J. E. Dickerson, and R. F. Heck, J. Org. Chem., 1978, 43, 5018; CrossRef F. G. Stakem and R. F. Heck, J. Org. Chem., 1980, 45, 3584. CrossRef
25. M. R. Fielding, R. Grigg, and C. J. Urch, Chem. Commun., 2000, 2239; CrossRef J. Anwar, M. R. Fielding, R. Grigg, V. Sridharan, and C. J. Urch, J. Organomet. Chem., 2006, 691, 1476. CrossRef
26. E. Piers and R. D. Tillyer, Can. J. Chem., 1996, 74, 2048. CrossRef
27. C. Pellegrim, M. Eber, and H.-J. Borschberg, Helv. Chim. Acta, 1996, 79, 151. CrossRef
28. F. Y. Miyake, K. Yakushijin, and D. A. Horne, Org. Lett., 2004, 6, 711. CrossRef
29. J. D. White, Y. Li, and D. C. Ihle, J. Org. Chem., 2010, 75, 3569. CrossRef
30. W. Cabri and I. Candiani, Acc. Chem. Res., 1995, 28, 2; CrossRef I. P. Beletskaya and A. V. Cheprakov, Chem. Rev., 2000, 100, 3009; CrossRef J. T. Link Org. React., 2002, 60, 157.
31. H. Qin, N. Yamagiwa, S. Matsunaga, and M. Shibasaki, J. Am. Chem. Soc., 2006, 128, 1611; CrossRef H. Qin, N. Yamagiwa, S. Matsunaga, and M. Shibasaki Chem. Asian J., 2007, 2, 150. CrossRef
32. H. Wei, G. Qian, Y. Xia, K. Li, Y. Li, and W. Li, Eur. J. Org. Chem., 2007, 4471; CrossRef X. Cheng, Y. Xia, H. Wei, B. Xu, C. Zhang, Y. Li, G. Qian, X. Zhang, K. Li, and W. Li, Eur. J. Org. Chem., 2008, 1929.
33. I. Beletskaya and C. Moberg, Chem. Rev., 2006, 106, 2320. CrossRef
34. Y. Sato, N. Saito, and M. Mori, Chem. Lett., 2002, 38, 18; CrossRef N. Saito, M. Mori, and Y. Sato, J. Organomet. Chem., 2007, 692, 460. CrossRef
35. C.-M. Yu, J. Youn, S.-K. Yoon, and Y.-T. Hong, Org. Lett., 2005, 7, 4507. CrossRef
36. M. Tobisu, Y. Kita, and N. Chatani, J. Am. Chem. Soc., 2006, 128, 8152; CrossRef T. Miura, Y. Takahashi, and M. Murakami, Org. Lett., 2008, 9, 1743. CrossRef
37. Y. Obora, Y. Tsuji, and T. Kawamura, J. Am. Chem. Soc., 1993, 115, 10414; CrossRef Y. Obora, Y. Tsuji, and T. Kawamura, J. Am. Chem. Soc., 1995, 117, 9814; CrossRef F.-Y. Yang, M. Shanmugasundaram, S.-Y. Chuang, P.-J. Ku, M.-Y. Wu, and C.-H. Cheng, J. Am. Chem. Soc., 2003, 125, 12576. CrossRef
38. D. R. Adams and S. P. Bhatnagar, Synthesis, 1977, 661; CrossRef T. Mukaiyama and M. Murakami, Synthesis, 1987, 1043. CrossRef
39. L. E. Overman and D. A. Watson, J. Org. Chem., 2006, 71, 2587. CrossRef
40. While the mechanism of the palladium-catalyzed carbosilylation has not been disclosed, we assumed the two plausible mechanisms. One is shown in Scheme 8. The other plausible pathway would commence with the oxidative addition of the carbamoyl chloride to a palladium catalyst. Insertion into the diene moiety would be followed by transmetallation with Me3SiSiMe3 and reductive elimination to give the product. We previously reported (refs. 19d, 20 and 21) that the addition of PPh3 was beneficial for the oxidative addition of the carbamoyl chloride to palladium, however, the addition of PPh3 in this case proved to be detrimental to the cyclization (Table 4, entries 8 and 9), and it was therefore assumed that the reaction proceeded via a bismetallative process..
41. A. Numata, C. Takahashi, Y. Ito, T. Takada, K. Kawai, Y. Usami, E. Matsumura, M. Imachi, T. Ito, and T. Hasegawa, Tetrahedron Lett., 1993, 34, 2355; CrossRef H. Hayashi, H. Matsumoto, and K. Akiyama, Biosci. Biotechnol. Biohem., 2004, 68, 753; CrossRef R. Jadulco, R. A. Edrada, R. Ebel, A. Berg, K. Schaumann, V. Wray, K. Steube, and P. Proksch, J. Nat. Prod., 2004, 67, 78; CrossRef P. W. Dalsgaard, J. W. Blunt, M. H. G. Munro, J. C. Frisvad, and C. Christophersen, J. Nat. Prod., 2005, 68, 258; CrossRef B. Andersen, J. Smedsgaard, and J. C. Frisvad, J. Agric. Food Chem., 2004, 52, 2421; CrossRef L. J. Wigley, P. G. Mantle, and D. A. Perry, Phytochemistry, 2006, 67, 561. CrossRef
42. S. M. Verbitski, C. L. Mayne, R. A. Davis, G. P. Concepcion, and C. M. Ireland, J. Org. Chem., 2002, 67, 7124. CrossRef
43. J. S. Carlé and C. Christophersen, J. Org. Chem., 1981, 46, 3440. CrossRef
44. N. Koyama, Y. Inoue, M. Sekine, Y. Hayakawa, H. Homma, S. Ōmura, and H. Tomoda, Org. Lett., 2008, 10, 5273. CrossRef
45. A. Hirano, Y. Iwai, R. Masuma, K. Tei, and S. Ōmura, J. Antibiot., 1979, 32, 781. CrossRef
46. C. Bunders, J. Cavanagh, and C. Melander, Org. Biomol. Chem., 2011, 9, 5476. CrossRef
47. T. Ishida, C. Tsukano, and Y. Takemoto, Chem. Lett., 2012, 41, 44. CrossRef
48. T. Ishida and Y. Takemoto, Tetrahedron, 2013, 69, 4517. CrossRef
49. T. Kawasaki, R. Terashima, K. Sakaguchi, H. Sekiguchi, and M. Sakamoto, Tetrahedron Lett., 1996, 37, 7525; CrossRef Y. Jiang, J. Zhao, and L. Hu, Tetrahedron Lett., 2002, 43, 4589; CrossRef Y. A. O. Barbosa, D. J. Hart, and N. A. Magomedov, Tetrahedron, 2006, 62, 8748; CrossRef A. Sabahi, A. Novikov, and J. D. Rainier, Angew. Chem. Int. Ed., 2006, 45, 4317; CrossRef M. Ohno, T. F. Spande, and B. Witkop, J. Am. Chem. Soc., 1968, 90, 6521. CrossRef
50. D. Aburano, T. Yoshida, N. Miyakoshi, and C. Mukai, J. Org. Chem., 2007, 72, 6878. CrossRef
51. B. Witulski, C. Alayrac, and L. Tevzadze-Saeftel, Angew. Chem. Int. Ed., 2003, 42, 4257. CrossRef
52. M. K. G. Mekhael and H. Heimgartner, Helv. Chim. Acta, 2003, 86, 2805. CrossRef
53. D. Dhanak, S. Neidle, and C. B. Reese, Tetrahedron Lett., 1985, 26, 2017. CrossRef
54. J. R. Fuchs and R. L. Funk, J. Am. Chem. Soc., 2004, 126, 5068. CrossRef
55. Y. Matsuda, M. Kitajima, and H. Takayama, Org. Lett., 2008, 10, 125. CrossRef
56. A. Williams and I. T. Ibrahim, Chem. Rev., 1981, 81, 589. CrossRef
57. W. Ahrens and A. Berndt, Tetrahedron Lett., 1974, 15, 3741; CrossRef G. Brunton, J. F. Taylor, and K. U. Ingold, J. Am. Chem. Soc., 1976, 98, 4879. CrossRef
58. J. L. Namy, P. Girard, and H. B. Kagan, New J. Chem., 1977, 1, 5.
59. G. A. Molander and C. R. Harris, Chem. Rev., 1996, 96, 307; CrossRef K. Gopalaiah and H. B. Kagan, New J. Chem., 2008, 32, 607. CrossRef
60. D. J. Edmonds, D. Johnston, and D. J. Procter, Chem. Rev., 2004, 104, 3371; CrossRef K. C. Nicolaou, S. P. Ellery, and J. S. Chen, Angew. Chem. Int. Ed., 2009, 48, 7140. CrossRef
61. J. L. Namy, J. Souppe, and H. B. Kagan, Tetrahedron Lett., 1983, 24, 765; CrossRef S. Fukuzawa, A. Nakanishi, T. Fujinami, and S. Sakai, Chem. Commun., 1986, 624; CrossRef T. Imamoto and S. Nishimura, Chem. Lett., 1990, 19, 1141; CrossRef J. Inanaga, Y. Handa, T. Tabuchi, K. Otsubo, M. Yamaguchi, and T. Hanamoto, Tetrahedron Lett., 1991, 32, 6557; CrossRef N. Taniguchi T. Hata, and M. Uemura, Angew. Chem. Int. Ed., 1999, 38, 1232; CrossRef Y. H. Kim, H. S. Park, and D. W. Kwon, Synth. Commun., 1998, 28, 4517; CrossRef J. M. Ready, S. E. Reisman, M. Hirata, M. M. Weiss, K. Tamaki, T. V. Ovaska, and J. L. Wood, Angew. Chem. Int. Ed., 2004, 43, 1270.
62. M. Deng, Y. Yao, Y. Zhang, and Q. Shen, Chem. Commun., 2004, 2742. CrossRef
63. T. Saito, K. Tsuda, and Y. Saito, Tetrahedron Lett., 1996, 37, 209. CrossRef
64. T. Saito, H. Ohmori, E. Furuno, and S. Motoki, Chem. Commun., 1992, 22; CrossRef P. Molina, M. Alajarín, A. Vidal, and P. Sánchez-Andrada, J. Org. Chem., 1992, 57, 929. CrossRef
65. J. Inanaga, M. Ishikawa, and M. Yamaguchi, Chem. Lett., 1987, 16,1485. CrossRef
66. R. S. Miller, J. M. Sealy, M. Shabangi, M. L. Kuhlman, J. R. Fuchs, and R. A. Flowers II, J. Am. Chem. Soc., 2000, 122, 7718; CrossRef F. Machrouhi, B. Hmann, J. L. Namy, and H. B. Kagan, Synlett, 1996, 633. CrossRef
67. J. R. Fuchs and R. L. Funk, J. Am. Chem. Soc., 2004, 126, 5068; CrossRef S. L. Crawley and R. L. Funk, Org. Lett., 2003, 5, 3169. CrossRef
68. H. Wu, F. Xue, X. Xiao, and Y. Qin, J. Am. Chem. Soc., 2010, 132, 14052; CrossRef J. Yang, H. Song, X. Xiao, J. Wang, and Y. Qin, Org. Lett., 2006, 8, 2187; CrossRef H. Wu, X. Xiao, and Y. Qin, Synlett, 2011, 907.
69. J. A. May, R. K. Zeidan, and B. M. Stoltz, Tetrahedron Lett., 2003, 44, 1203; CrossRef S.-J. Han, F. Vogt, S. Krishnan, J. A. May, M. Gatti, S. C. Virgil, and B. M. Stoltz, Org. Lett., 2014, 16, 3316. CrossRef
70. G. D. Artman III and S. M. Weinreb, Org. Lett., 2003, 5, 1523; CrossRef J. H. Seo, G. D. Artman III, and S. M. Weinreb, J. Org. Chem., 2006, 71, 8891; CrossRef M. A. Evans, J. R. Sacher, and S. M. Weinreb, Tetrahedron, 2009, 65, 6712. CrossRef
71. A. Sabahi, A. Novikov, and J. D. Rainier, Angew. Chem. Int. Ed., 2006, 45, 4317. CrossRef
72. B. M. Trost and Y. Zhang, Chem. Eur. J., 2011, 17, 2916.
73. Y. Du, H. Wu, H. Song, Y. Qin, and D. Zhang, Chin. J. Chem., 2012, 30, 1970. CrossRef
74. A. W. Schammel, G. Chiou, and N. K. Garg, Org. Lett., 2012, 14, 4556. CrossRef
75. H. Zhang, L. Hong, H. Kang, and R. Wang, J. Am. Chem. Soc., 2013, 135, 14098. CrossRef
76. M. J. Sharp and V. Snieckus, Tetrahedron Lett., 1985, 26, 5997. CrossRef
77. C. B. Brain and T. Brunton, Tetrahedron Lett., 2002, 43, 1893; CrossRef M. A. McGowan, C. Z. McAvoy, and S. L. Buchwald, Org. Lett., 2012, 14, 3800. CrossRef
78. C. Tsukano, M. Okuno, and Y. Takemoto, Angew. Chem. Int. Ed., 2012, 51, 2763; CrossRef T. Nanjo, C. Tsukano, and Y. Takemoto, Org. Lett., 2012, 14, 4270; CrossRef C. Tsukano, M. Okuno, and Y. Takemoto, Chem. Lett., 2013, 42, 753; CrossRef T. Nanjo, S. Yamamoto, C. Tsukano, and Y. Takemoto, Org. Lett., 2013, 15, 3754; CrossRef T. Ishida, H. Ikota, K. Kurahashi, C. Tsukano, and Y. Takemoto, Angew. Chem. Int. Ed., 2013, 52, 10204; CrossRef T. Nanjo, C. Tsukano, and Y. Takemoto, Synlett, 2014, 25, 1473; CrossRef C. Tsukano, M. Okuno, H. Nishiguchi, and Y. Takemoto, Adv. Synth. Catal., 2014, 356, 1533. CrossRef