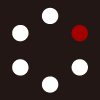
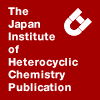
HETEROCYCLES
An International Journal for Reviews and Communications in Heterocyclic ChemistryWeb Edition ISSN: 1881-0942
Published online by The Japan Institute of Heterocyclic Chemistry
e-Journal
Full Text HTML
Received, 19th November, 2014, Accepted, 15th December, 2014, Published online, 7th January, 2015.
DOI: 10.3987/COM-14-13131
■ Further Investigation of Pyranone Activation
Justin A. Simanis, Christian R. Zwick, Erica L. Woodall, John R. Goodell, and T. Andrew Mitchell*
Campus Box 4160, Department of Chemistry, Illinois State University, Normal, Illinois 61790-4160, U.S.A.
Abstract
As part of our ongoing investigation into the activation parameters of pyranones to the corresponding oxidopyryliums, various means were investigated. Screening of acid mediators revealed limited efficiency, with HCl providing the best result. Additional acid-mediated and microwave-assisted studies provided evidence that oxocarbenium formation is a key mechanistic event thwarting formation of oxidopyrylium intermediates. A µW-assisted exchange process was discovered allowing for the rapid synthesis of potentially useful alkoxypyranones.Although oxidopyrylium-alkene [5+2] cycloaddition reaction1 are used extensively to access biologically active natural products2 containing a diverse range of bridged polycyclic ether substitution patterns, relatively little is known about the requisite activation parameters.3 The most common method to generate oxidopyrylium intermediates is base-mediated activation of acetoxypyranones.4 However, acylation of sterically hindered hydroxypyranone precursors has proven to be problematic5 since tertiary hydroxypyranones are known to undergo ring fragmentation upon exposure to amine bases required for acylation.6 Given this limitation of acetoxypyranone substitution at the acetal carbon,7 we sought to explore tertiary hydroxypyranone substrates in greater detail toward oxidopyrylium activation and subsequent [5+2] cycloaddition. An interesting solution demonstrated by Magnus involved the activation of a tertiary hydroxypyranone directly to an oxidopyrylium by exposure to trifluoroacetic acid.5b Except for an earlier example by Sammes that was likely a result of thermal rather than acid-activation,4b to our knowledge this TFA-mediated case5b is the sole example of acid-mediated hydroxypyranone activation to an oxidopyrylium intermediate. Intrigued by this potential alternative to base-mediated activation, we sought to explore this mode of activation en route to novel oxidopyrylium-alkene [5+2] cycloadditions. Intramolecular cycloadditions were utilized as model reactions due to an inherent entropic advantage that increases the rate of cycloaddition thus enhancing the probability of intercepting the proposed transient oxidopyrylium intermediate.4b Although acid-mediated activation gave poor conversion of desired cycloadduct, the insight gleaned shed light on acid-mediated,5b microwave-assisted,8 and base-mediated activation.9 Herein, we report these results and a µW-assisted formation of alkoxypyranones (Scheme 1).
Hydroxypyranone 1a was synthesized as a mixture of diastereomers (dr 2:1) in two steps. Acetoxypyranone 1b was prepared by treatment of 1a with acetyl chloride and pyridine. Flash column chromatography delivered diastereomerically pure anti- and syn-1b. The major acetoxypyranone, anti-1b (>19:1), was utilized to probe the mechanism of activation (vide infra). Reaction of hydroxypyranone 1a with various acids provided multiple outcomes (Table 1). AcOH provided only recovered starting material (entry 1), whereas HCl (entry 2) and CSA (entry 3) afforded minimal cycloadduct 2 along with by-products 3 and 4.1 Perchloric and triflic acids (entries 4 and 5) led to decomposition of starting material. Alternatives such as HCl salts of amines and Lewis acids (not shown) showed little promise to afford cycloadduct 2. In similar reactions with anti-1b, hydroxypyranone 1a was detected by 1H NMR analysis of crude reaction mixtures thus signifying its potential as an intermediate en route to cycloadduct 2. Consequently, our next objective was to ascertain whether acetoxypyranone anti-1b, in the presence of acid and water, was converted to hydroxypyranone 1a via an oxocarbenium intermediate2 or through simple hydrolysis. Thus, we screened various conditions utilizing high concentration of nucleophilic solvent in order to capture potential transient oxocarbenium intermediates. We found that anti-1b (dr >19:1) was readily converted to 1a (dr ~2:1) upon exposure to a range of acids and high concentration of H2O (Table 2, entries 1-4). Similar acidic conditions provided methoxypyranone 1c (dr ~1:1),10 albeit with lower yields presumably due to side reactions of the enone moiety (entries 5-8). The formation of acetal 1c from anti-1b provides critical information1 since it is incapable of forming as a result of hydrolysis. This supports oxocarbenium formation as the first mechanistic event in acid-mediated pyranone activation since nucleophilic attack at sp2-hybridized oxocarbenium intermediates would be expected to provide a mixture of diastereomers, while hydrolysis would maintain stereochemical fidelity. Interestingly, the diastereomeric ratio of hydroxypyranone 1a produced via acid-mediated exchange was similar to that observed when synthesized by previously reported methods.9 Analogous acid-mediated reactions of hydroxypyranone 1a led to decomposition of starting material. These results provide evidence that oxocarbenium formation plays a crucial role in the conversion to pyranones 1a and 1c.
Based on acid-mediated exchange results that suggest initial oxocarbenium en route to oxidopyrylium generation, an analogous study was conducted to determine if µW-assisted conditions would proceed in similar fashion. Toward this end, pyranones 1a and anti-1b were subjected to microwave irradiation in MeCN:MeOH (1:1) for ten minutes (Table 3). Heating to 100 °C was insufficient to obtain significant conversion of either 1a or 1b to methoxypyranone 1c (entries 1, 5). As compared to anti-1b, hydroxypyranone 1a was more readily converted to 1c at elevated temperatures (entries 2-3, 6-7). In contrast, upon heating to 160 °C and isolation of methoxypyranone 1c, greater efficiency with anti-1b was observed as compared to 1a (entries 4, 8). Similar to the acid-mediated activation, isolation of a diastereomeric mixture suggests that oxocarbenium intermediates are formed in these reactions. In order to explore the scope of this exchange process, we attempted to substitute various alcohols (Table 4). Upon increasing the concentration (0.1 → 1 M), moderate yield was obtained with just two equivalents of MeOH (entry 1). Isopropanol afforded a similar result (entry 2) and other primary alcohols were successful (entries 3-5). In all cases, minimal cycloadduct 2 was observed further demonstrating the lack of efficiency of oxocarbenium conversion to oxidopyrylium. Other nucleophiles (not shown) were unproductive: trifluoroethanol afforded low yield and N-hydroxyphthalimide gave decomposition.
Taken together, these results stand in stark contrast to previous findings utilizing base-mediated activation (Scheme 2).9 Specifically, we used kinetic isotope effects to demonstrate that abstraction of the α-hydrogen is involved in the rate-determining step of oxidopyrylium generation. Elimination of the acetoxy from dienolate 5 provides oxidopyrylium 6, which undergoes [5+2] cycloaddition to tricyclic ether 2. In contrast, both acid-mediated and microwave-assisted activation of pyranones 1a and 1b (vide supra) suggest that oxocarbenium 7 is formed prior to oxidopyrylium 6. Although not a direct correlation, inference from the calculated ground-state conformation of oxocarbenium 713 suggests that an energetically unfavorable deprotonation of the α-proton10 may explain the fact that oxocarbenium 7 inefficiently progresses toward oxidopyrylium 6. Hydroxypyranones utilized in previous examples4b,5b contain structural features that likely explain the increased yields as compared to our model system (vide supra). In these cases, the alkene tether is appended to the same carbon as the hydroxyl group. Consequently, it seems plausible that two α-hydrogens may allow for a lower activation energy barrier of deprotonation due to increased orbital overlap with the adjacent ketone toward oxidopyrylium formation.
Activation parameters of various pyranones were investigated. Screening of acid mediators in a model reaction revealed limited cycloaddition with multiple by-products. Multiple experiments utilized both acid-mediated and microwave-assisted methods to provide evidence suggestive of oxocarbenium formation as the initial mechanistic event toward the generation of oxidopyrylium intermediates. This is contrary to our previous results with base-mediated processes which suggest initial deprotonation of the α-proton en route to oxidopyrylium intermediates. Both of these studies, taken together, aid our understanding of the activation parameters of acetoxypyranones, which will be further investigated synthetically and computationally. As a more complete description of these parameters emerges, this will inevitably lead to advances toward novel cycloaddition processes.
EXPERIMENTAL
Pyranone 1c: Prepared according to the general procedure with anti-1b (208 mg, 0.927 mmol), MeOH (75 µL, 1.85 mmol), and MeCN (925 µL). Purification by flash column chromatography (hexanes:EtOAc 90:10) delivered anti-1c as a white solid (35 mg, 0.18 mmol, 20%, dr >19:1), and anti-1c/syn-1c mixture as a yellow oil (89 mg, 0.45 mmol, 49%, dr 1.4:1.0). Spectral data matched that obtained from the alternative conditions toward pure diastereomers of methoxypyranone 1c (vide supra).
Pyranone 1d: Prepared according to the general procedure with anti-1b (200 mg, 0.892 mmol), iPrOH (107 mg, 1.78 mmol), and MeCN (890 µL). Purification by flash column chromatography (hexanes:EtOAc 90:10) delivered anti-1d as a pale yellow oil (49 mg, 0.22 mmol, 25%, dr >19:1), anti-1d/syn-1d mixture as a yellow oil (76 mg, 0.34 mmol, 38%, dr 1.7:1.0), and anti-1d/syn-1d mixture as a yellow oil (11 mg, 0.05 mmol, 5%, dr 0.13:1.0); Characterization data for anti-1d: Rƒ = 0.49 (hexanes:EtOAc 85:15); 1H-NMR (500 MHz, CDCl3) δ 6.80 (dd, J = 10.2, 3.6 Hz, 1H), 6.06 (dd, J = 10.2, 0.6 Hz, 1H), 5.85-5.77 (m, 1H), 5.30 (dd, J = 3.6, 0.6 Hz, 1H), 5.03-4.99 (m, 1H), 4.97-4.94 (m, 1H), 4.44 (dd, J = 8.4, 3.6 Hz, 1H), 4.05 (qq, J = 6.3, 6.1 Hz, 1H), 2.15-2.05 (m, 2H), 2.01-1.95 (m, 1H), 1.72-1.64 (m, 1H), 1.60-1.49 (m, 2H), 1.27 (d, J = 6.3 Hz, 3H), 1.23 (d, J = 6.1 Hz, 3H); 13C-NMR (400 MHz, CDCl3) δ 196.9, 144.0, 138.4, 127.5, 114.7, 91.3, 73.8, 70.8, 33.5, 29.1, 24.5, 23.3, 21.8; IR (film) νmax 1700, 1049 cm-1; ESI-HRMS calculated for C13H20O3 [M+Li]+ 231.1567, found 231.1560.
Pyranone 1e: Prepared according to the general procedure with anti-1b (224 mg, 1.00 mmol, 1.0 equiv.), 5-hexen-1-ol (93.5 mg, 2.00 mmol, 2.0 equiv.), and MeCN (925 µL). Purification by flash column chromatography (hexanes:Et2O 85:15) delivered anti-1e as a pale yellow oil (112 mg, 0.42 mmol, 42%, dr >19:1), and anti-1e/syn-1e mixture as a yellow oil (54 mg, 0.20 mmol, 20%, dr 0.21:1.0); Characterization data for anti-1e: Rƒ = 0.26 (hexanes:Et2O 85:15); 1H-NMR (500 MHz, CDCl3) δ 6.83 (dd, J = 10.2, 3.5 Hz, 1H), 6.07 (dd, J = 10.2, 0.6 Hz, 1H), 5.86-5.76 (m, 2H), 5.19 (dd, 3.5, 0.6 Hz, 1H), 5.04-4.99 (m, 2H), 4.98-4.95 (m, 2H), 4.40 (dd, J = 8.4, 3.6 Hz, 1H), 3.85-3.80 (m, 1H), 3.6-3.56 (m, 1H), 2.15-2.04 (m, 4H), 2.02-1.95 (m, 1H), 1.68-1.46 (m, 7H); 13C-NMR (400 MHz, C6D6) δ 195.4, 142.9, 138.31, 138.30, 127.1, 114.6, 114.5, 93.1, 73.8, 68.7, 33.5, 33.4, 29.13, 29.05, 25.4, 24.5; IR (film) νmax 1700, 1034 cm-1; ESI-HRMS calculated for C16H24O3 [M+Li]+ 271.1880, found 271.1892.
Pyranone 1f: Prepared according to the general procedure with anti-1b (206 mg, 0.917 mmol), ethyl glycolate (191 mg, 1.83 mmol), and MeCN (915 µL). Purification by flash column chromatography (hexanes:EtOAc 85:15) delivered anti-1f/syn-1f mixture (111 mg) contaminated with cycloadduct 2 (13 mg) as a pale yellow oil (calc. 98 mg, 0.37 mmol, 40%, dr 1.49:1.0) and syn-1f as a yellow oil (11 mg, 0.045 mmol, 4%, dr >19:1); Characterization data for syn-1f: Rƒ = 0.21 (hexanes:EtOAc 85:15); 1H-NMR (400 MHz, CDCl3) δ 6.98 (dd, J = 10.4, 2.1 Hz, 1H), 6.15 (dd, J = 10.4, 1.5 Hz, 1H), 5.84-5.74 (m, 1H), 5.46-5.45 (m, 1H), 5.04-4.99 (m, 1H), 4.98-4.94 (m, 1H), 4.37 (d, J = 16.4 Hz, 1H), 4.32 (d, J = 16.4 Hz, 1H), 4.27-4.21 (m, 2H), 4.08-4.04 (m, 1H), 2.15-2.01 (m, 2H), 1.97-1.78 (m, 2H), 1.65-1.48 (m, 2H), 1.30 (dd, J = 7.2, 7.1 Hz, 3H); 13C-NMR (400 MHz, CDCl3) δ 196.0, 169.7, 145.4, 138.2, 128.7, 114.9, 95.0, 79.0, 64.7, 61.2, 33.4, 31.1, 24.7, 14.2; IR (film) νmax 1751, 1699, 1063 cm-1; ESI-HRMS calculated for C14H20O5 [M+Li]+ 275.1466, found 275.1454.
Pyranone 1g: Prepared according to the general procedure with anti-1b (210 mg, 0.936 mmol), 2-allyloxyethanol (191 mg, 1.87 mmol) and MeCN (935 µL). Purification by flash column chromatography (hexanes:EtOAc 90:10) delivered anti-1g/syn-1g mixture (181 mg) contaminated with cycloadduct 2 (18 mg) as a pale yellow oil (calc. 163 mg, 0.61 mmol, 65%, dr 3.7:1.0) and syn-1g as a yellow oil (19 mg, 0.07 mmol, 8%, dr >19:1); Characterization data for syn-1g: Rƒ = 0.15 (hexanes:EtOAc 90:10); 1H-NMR (400 MHz, CDCl3) δ 6.92 (dd, J = 10.3, 1.9, 1H), 6.12 (dd, J = 10.3, 1.7, 1H), 5.97-5.87 (m, 1H), 5.86-5.76 (m, 1H), 5.40-5.39 (m, 1H), 5.31-5.26 (m, 1H), 5.21-5.18 (m, 1H), 5.05-4.98 (m, 1H), 4.97-4.94 (m, 1H), 4.05-4.01 (m, 4H), 3.85-3.79 (m, 1H), 3.67-3.64 (m, 2H), 2.14-2.03 (m, 2H), 2.01-1.77 (m, 2H), 1.68-1.48 (m, 2H); 13C-NMR (400 MHz, CDCl3) δ 196.4, 146.6, 138.4, 134.6, 128.6, 117.3, 114.8, 96.0, 78.9, 72.3, 69.2, 68.1, 33.4, 30.7, 24.7; IR (film) νmax 1700, 1051 cm-1; ESI-HRMS calculated for C15H22O4 [M+H]+ 267.1596, found 267.1581.
ACKNOWLEDGEMENTS
Acknowledgment is made to the Donors of the American Chemical Society Petroleum Research Fund (PRF #52391-UNI1). Funding from Illinois State University is gratefully acknowledged.
References
1. N. Nishiwaki, ‘Methods and Applications of Cycloaddition Reactions in Organic Synthesis,’ Wiley-VCH: Weinheim, 2014; CrossRef S. Kobayashi and K. A. Jørgensen, ‘Cycloaddition Reactions in Organic Chemistry,’ Wiley-VCH: Weinheim, 2002; W. Carruthers, ‘Cycloaddition Reactions in Organic Chemistry,’ Vol. 8 (Tetrahedron Organic Chemistry), Pergamon: Oxford, 1990.
2. M. J. Palframan and G. Pattenden, Chem. Commun., 2014, 50, 7223; CrossRef K. E. O. Ylijoki and J. M. Stryker, Chem. Rev., 2013, 113, 2244; CrossRef M. Guangjian, H. Yuan, Y, Gu, W. Chen, L. W. Chung, and C. C. Li, Angew. Chem. Int. Ed., 2014, 53, 11051; J.-H. Sohn, Bull. Korean Chem. Soc., 2014, 35, 23; CrossRef M. Zhang, N. Liu, and W. Tang, J. Am. Chem. Soc., 2013, 135, 12434; CrossRef F. Rodier, J.-L. Parrain, G. Chouraqui, and F. L. Commeiras, Org. Biomol. Chem., 2013, 11, 4178. CrossRef
3. V. Singh, U. M. Krishna, Vikrant, and G. K. Trivedi, Tetrahedron, 2008, 64, 3405. CrossRef
4. J. B. Hendrickson and J. S. Farina, J. Org. Chem., 1980, 45, 3359; CrossRef P. G. Sammes and L. J. Street, J. Chem. Soc., Chem. Commun., 1982, 1056. CrossRef
5. K. A. Marshall, A. K. Mapp, and C. H. Heathcock, J. Org. Chem., 1996, 61, 9135; CrossRef P. Magnus and L. Shen. Tetrahedron, 1999, 55, 3553. CrossRef
6. W. H. Miles, P. G. Gildner, Z. Ahmed, and E. M. Cohem, Synthesis, 2010, 3977. CrossRef
7. P. A. Wender, F. C. Bi, N. Buschmann, F. Gosselin, C. Kan, J. M. Kee, and H. Ohmura, Org. Lett., 2006, 8, 5373; CrossRef N. Z. Burns, M. R. Witten, and E. N. Jacobsen, J. Am. Chem. Soc., 2011, 133, 14578; CrossRef P. Wender, C. D. Jesudason, H. Nakahira, N. Tamura, A. L. Tebbe, and Y. Ueno, J. Am. Chem. Soc., 1997, 119, 12976; CrossRef R. M. Adlington, J. E. Baldwin, A. V. W. Mayweg, and G. J. Pritchard, Org. Lett., 2002, 17, 3009. CrossRef
8. P.-K. Chen, M. R. Rosana, G. B. Dudley, and A. E. Stiegman, J. Org. Chem., 2014, 79, 7425; CrossRef C. O. Kappe, Angew. Chem. Int. Ed., 2004, 43, 6250. CrossRef
9. E. L. Woodall, J. A. Simanis, C. G. Hamaker, J. R. Goodell, and T. A. Mitchell, Org. Lett., 2013, 15, 3270. CrossRef
10. See Supporting Information for further details.
11. L. Bohe and D. Crich, Carbohydr. Res., 2014, Article in Press.
12. J. Ren, Y. Liu, L. Song, and R. Tong, Org. Lett., 2014, 16, 2986; CrossRef M. D. Burke, E. M. Berger, and S. L. Schreiber, J. Am. Chem. Soc., 2004, 126, 14095; CrossRef X. Li and J. Zhu, J. Carbohydr. Chem., 2012, 31, 284; CrossRef J. P. M. Nunes, L. F. Veiros, P. D. Vza, C. A. M. Afonso, and S. Caddick, Tetrahedron, 2011, 67, 2779; CrossRef Y. Yoshimura, H. Shimizu, H. Hinou, and S. I. Nishimura, Tetrahedron Lett., 2005, 46, 4701; CrossRef A. C. Comely, R. Eelkema, A. J. Minnaard, and B. L. Feringa, J. Am. Chem. Soc., 2003, 125, 8714; CrossRef H. Takao, N. Osaki, and N. Yasudomi, U. S. Patent 4742078, May 3, 1988.
13. Conformational analysis (Spartan ’02, Wavefunction, Inc.) using MMFF94 force field, optimization (Gaussian ‘03), DTF-B3LYP, 6-31g(d,p), Gaussian, Inc.: Wallingford CT, 2004.