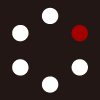
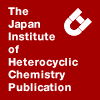
HETEROCYCLES
An International Journal for Reviews and Communications in Heterocyclic ChemistryWeb Edition ISSN: 1881-0942
Published online by The Japan Institute of Heterocyclic Chemistry
e-Journal
Full Text HTML
Received, 7th January, 2015, Accepted, 16th February, 2015, Published online, 24th February, 2015.
DOI: 10.3987/REV-15-818
■ Transition Metal-Catalyzed Intramolecular Cyclization of Propargyl Alcohols and Their Derivatives for the Synthesis of Highly Substituted Five-Membered Oxygen Heterocycles
Masahiro Egi* and Shuji Akai*
Synthetic Medicinal Chemistry, Graduate School of Pharmaceutical Sciences, Osaka University, 1-6 Yamadaoka, Suita, Osaka 565-0871, Japan
Abstract
The transition metal-catalyzed intramolecular cyclization of propargyl alcohols and their derivatives has been widely utilized in the synthesis of five-membered oxygen heterocycles such as furans, hydrofurans, and furanones. Prerequisites for the efficient transformations into highly substituted target compounds include the regioselectivity of the cyclization step and the chemoselectivity of the transition metal-mediated activation of substrates. This review documents recent progress on the title reactions by categorizing the initial activation modes of substrates.CONTENTS
1. Introduction
2. Nucleophilic cyclization triggered by acetylene activation
3. Cascade processes initiated by rearrangements
3.1 Participation of both the acetylene and propargylic oxygen-substituent moieties in ring formation
3.2 Participation of either the acetylene or the propargylic oxygen-substituent moiety in ring formation
4. Cascade processes initiated by propargylic elimination
5. Other types of ring formation
6. Conclusion
7. References
1. INTRODUCTION
Five-membered oxygen-containing heterocycles constitute one of the most fundamental compounds in organic chemistry.1 Among these families, the aromatic compounds, i.e. furans, play a central role in widespread applications. The furan skeleton is found abundantly in natural products as well as in pharmaceuticals, which demonstrates the broad spectrum of biological activities such as antibacterial, insecticidal, antidepressant, and anti-inflammatory activities.1a,2 More recently, in the field of material science, the oligomers and polymers of furans have shown promise for application in electronic devices.3 Furans and their derivatives have also been employed as versatile building blocks in organic synthesis due to their wide range of reactivities. The reduced forms of furans (2,3-dihydrofurans, 2,5-dihydrofurans, and tetrahydrofurans) as well as the oxidized forms, such as the keto-enol tautomers of furan-2(5H)-ones (α,β-unsaturated lactones or butenolides), and those of furan-3(2H)-ones, also have great potential in biological, medicinal, and pharmaceutical sciences, and each compound exhibits characteristic reactivities which are different from those of furans. The usefulness of these five-membered oxygen heterocycles has stimulated organic chemists to develop effective and powerful synthetic methods for their preparation.4 In the case of furans, in addition to the traditional synthesis by either the Paal–Knorr method or the Feist–Bénary method, the transition metal-catalyzed intramolecular cyclization of acyclic precursors such as allenyl ketones, alkynyl ketones, enynes, diynes, and alkynyl cyclopropyl ketones, enable the direct synthesis of furans bearing diverse functional groups.5 These methods have recently received an increasing amount of attention from the viewpoint of environmentally friendliness and atom efficiency. Metal catalysts, including copper, zinc, palladium, silver, and platinum, have been widely utilized for these ring closures.5
Propargyl alcohols and their derivatives have also attracted particular attention as versatile precursors in a range of organic syntheses.6 This is likely to be due to the fact that they are easily prepared by the alkynylation of carbonyl compounds, or by reduction of propargyl ketones, and also because they show various reactivities at two main reactive sites, namely the triple bond and the oxygen functional group. The triple bond is capable of coordinating to various transition metals due to its high electron density (type A interaction), and can undertake nucleophilic additions. The oxygen functional group can also coordinate to transition metals (type B interaction), which enhances the elimination of the oxygen functional group and can subsequently result in substitution with a variety of nucleophiles. In some cases, the activation of propargyl compounds is understood to be caused by the dual coordination of the triple bond and the oxygen functional group to a single transition metal (type C interaction). These three types of interactions are demonstrated in Figure 1.
Recently, we have developed a number of novel transition metal-catalyzed transformations of propargyl alcohols.7 These transitions include the direct catalytic deoxygenation into the corresponding alkynes via a type B interaction (eq. 1), the rapid 1,3-rearrangement of the hydroxyl group into a variety of α,β-unsaturated carbonyl compounds via a type C interaction (eq. 2), and rearrangement into the synthetically challenging (Z)-α,β-unsaturated ketones via a type C interaction (eq. 3).
We then applied the use of propargyl alcohol derivatives to the synthesis of five-membered oxygen-containing heterocycles via a transition metal-catalyzed intramolecular cyclization.8 In this review, we therefore document the transition metal-catalyzed intramolecular cyclizations of propargyl alcohols and their derivatives to give five-membered oxygen heterocycles, including details of our own research into the area. We have chosen to focus mainly on the discussion of the more recent literatures (since 2004), but some earlier transformations of importance are also introduced where appropriate. The contents of our review are classified into four sections based on various reaction modes (Figure 2): (a) nucleophilic cyclization triggered by acetylene activation; (b) rearrangement (or isomerization) of propargyl alcohol derivatives followed by cyclization; and (c) propargylic elimination followed by cyclization. Other types of reaction are also discussed, but are not outlined in Figure 2.
2. NUCLEOPHILIC CYCLIZATION TRIGGERED BY ACETYLENE ACTIVATION
Utimoto et al. reported a pioneering study into the transition metal-catalyzed intramolecular cyclization of propargyl diols to give substituted furans.9 A typical example is shown in Scheme 1, where the hydroxyl group at the homopropargylic position of diol 1 undergoes a 5-endo-dig addition to the acetylene moiety, activated by coordination to the palladium catalyst, followed by dehydration to give furan 4. In this case, the Meyer–Schuster rearrangement, one of the well-known reactions of propargyl alcohols,6e was not observed.
Inspired by this report, the intramolecular cyclization of propargyl diols has been extensively investigated by using a range of transition metals as follows. Miyashita et al. reported the AgNO3-catalyzed transformation of diols 5 into furans 6 in the synthetic studies of zoanthamine alkaloids, although the process was not discussed in any great detail.10 Later, Knight et al. reported a similar system where they employed a catalytic amount of AgNO3 immobilized on silica gel (Scheme 2).11 This catalytic system enabled the cyclization of 5 to take place at ambient temperature within a short reaction time.
Aponick et al. disclosed that a combination of catalytic amounts of [(o-biphenyl)(t-Bu)2P]AuCl and AgOTf was suitable for the cyclization of 5, albeit with precautions taken to exclude water by the use of molecular sieves (Scheme 3).12 In addition, they found that AuCl was active even in an open vessel.
We ourselves have also been active in the area of gold-catalyzed transformations of diols. We carried out the intramolecular cyclization of 5 with a combination of Ph3PAuCl and AgNTf2, further enhancing the efficiency of the reaction by using a catalytic system, which showed high reactivity even in the presence of water.8a Our developed methodology was widely applicable to a range of diols and afforded furans in high yields (Table 1). In particular, we found that propargyl alcohols bearing a terminal alkyne moiety were suitable for the preparation of furans (entries 6–8), while the aforementioned methods failed to deliver any products from these substrates. Moreover, this system was successfully applied to the multigram-scale synthesis of furans at high concentrations (0.8 M in toluene, entry 2). We also noted that anti-7 showed slightly higher reactivity than its syn-diastereoisomer, as outlined in Scheme 4.
Molybdenum- and ruthenium-based catalysts were used in the preparation of furans via the cyclization of diols bearing a terminal alkynyl group. For example, the McDonald group disclosed the cyclization of 9, induced by a stoichiometric amount of Mo(CO)6 in the presence of trimethylamine N-oxide (Scheme 5a).13 Following this, the Nishibayashi group developed a catalytic method using the methanethiolate-bridged diruthenium complex 13 (5 mol%) (Scheme 5b).14 It was proposed that these transformations proceeded via the formation of metal vinylidenes 10 and 14, which differed from the above-mentioned mechanism based on palladium, silver, and gold catalysis. The formation of ruthenium cumulenylidene 15, considered as a key intermediate, resulted in the cyclization of the remaining hydroxyl group to form the furan along with regeneration of the transition metal species.
As an extension of the intramolecular cyclization of propargyl diols, the cascade cyclization/coupling reaction was investigated, promoted by complexation of a palladium catalyst to propargyl diol 17 or 5. Utimoto et al. initially attempted the coupling reaction of the resultant palladium intermediate 20 with allyl halide 18 (Scheme 6a).9 The formation of the protonated side product 22 was suppressed by using an excess amount of epoxide as a proton scavenger to give the desired 3-allylfuran 21 preferentially. More recently, Gabriele et al. developed the synthesis of tetrasubstituted furans 24 by the palladium-catalyzed cascade cyclization/alkoxycarbonylation (Scheme 6b).15 They proposed that the 5-endo-dig cyclization occurred via intramolecular nucleophilic attack of the β-hydroxyl group to the palladium-activated
acetylene group (5→23), followed by alkoxycarbonylation/dehydration (23→24) in preference to protonation.
With the aim to reduce waste and to improve the applicability of these reactions, we synthesized the polystyrene-immobilized gold(I) catalysts 27a and 27b (Scheme 7), for the transformation of diols 5 into furans 6.8c The commercially available polystyrene-bound triphenylphosphine 25 was treated with Me2SAuCl in CH2Cl2, followed by the addition of either AgOTf or AgNTf2 in toluene to give 27a or 27b, respectively. This was, to the best of our knowledge, the first example of the preparation of an immobilized cationic gold(I) catalyst. The immobilized catalysts 27a and 27b afforded similar results in the cyclization of 5 (Scheme 8), although a longer reaction time was required compared to our catalyst combination of Ph3PAuCl and AgNTf2. The catalyst 27a was easily and quantitatively recovered by simple decantation, and was reused in seven further reactions without significant loss of reactivity (Table 2). We observed that during subsequent runs using the recycled catalyst, only a very small amount of gold species leached out from the polystyrene support. Moreover, 27a was suitable for use in a flow reactor system for the rapid and continuous production of 29 (Figure 3).
We then applied the intramolecular cyclization reaction of propargyl diols to the synthesis of unsaturated lactones. For this purpose, propargyl diols 30 bearing a phenoxy group at the acetylene terminus were designed based on our expectations of where the phenoxy moiety would enhance the reactivity of the acetylene moiety, and also of where it would work best as a leaving group. Using AgOTf, the intramolecular cyclization of 30 proceeded smoothly at room temperature to give α,β-unsaturated lactones 31 in good to excellent yields (Scheme 9).8d
This method is undoubtedly beneficial for the preparation of multisubstituted α,β-unsaturated lactones (R1, R2 ≠ H), whose structures are often presented as central structural elements in natural products. A plausible mechanism for this transformation is proposed in Scheme 10. The higher electron density of the phenoxyethynyl moiety of 30, as is evident from its resonance form 30ʹ, accelerates coordination of the acetylene moiety to the silver cation. Oxonium intermediate 32, generated in situ, is susceptible to nucleophilic attack even by sterically hindered tertiary alcohols (32→33). Subsequently, the donation of lone pair electrons from the ethereal oxygen atom results in the generation of the transient oxonium ion 34 and water. The final step involves the reaction of 34 with water to give 35, which subsequently releases phenol to give substituted unsaturated lactones 31.
At around the same time, Lamaty et al. reported the use of poly(ethylene glycol) (PEG) as an alternative reaction medium to the volatile organic solvents.16 The platinum- and gold-catalyzed reactions of 5 in PEG-3400 were conducted under microwave heating to afford a range of furans 6 in good yields (Scheme 11). PEG was thought to stabilize and encapsulate the metallic species, thus preventing aggregation and deactivation. This catalytic system allowed the reuse of these catalysts up to four times in subsequent
reactions, and it was found that the high reactivity of AuCl3 could be maintained by the presence of a trace amount of benzoquinone during subsequent reactions.
Pale et al. reported the AgOTf-catalyzed reaction of propargyl epoxides 36 with methanol (Scheme 12).17 In this reaction, the nucleophilic addition of methanol to 36 led to the opening of the epoxide ring, forming in situ propargyl diol intermediates 37 and 38. Regioisomers 37 and 38 showed different reactivities to the silver catalyst, and only 37 was converted into the desired furan product 6. The same group later disclosed that the use of Ph3PAuOTf resulted in the complete conversion of 36 to intermediates 37 and 38 at a faster rate than for the silver catalyst.17b In the case of gold catalysis, both 37 and 38 were consumed, albeit at different rates, leading to the formation of furans 6.
Without having to rely on the formation of propargyl diol intermediates, propargyl epoxides 36 showed high enough reactivity to form furans 41 and 6 by coordination to various transition metal catalysts, such as molybdenum, ruthenium, gold, and platinum (Scheme 13).18 The mechanisms for these transformations include direct attack of the oxygen atom of the epoxide ring on either a metal–vinylidene species 39 or metal–alkyne π-complex 42.
Moreover, Liang et al. reported the gold-catalyzed reaction of propargyl esters 44 bearing an epoxide moiety to give furans 49 (Scheme 14).19 This reaction involved the alkyne activation of 44 with gold catalysts to give 46, and the subsequent cascade endo-dig cyclization/nucleophilic attack to give intermediates 48. Protonation of the gold species and elimination of acetic acid resulted in conversion of 48 to 49. They reported that the use of AuCl3 (2 mol%) at room temperature, or HAuCl4·4H2O (2 mol%) at 60 °C was among the most suitable condition for the transformation. Screening of a range of external nucleophiles 45 revealed that even the sterically hindered alcohols could be successfully employed in this reaction.
Aside from the hydroxyl and epoxide groups, it was also observed that the carbonyl and active methylene groups were able to react with transition metal-coordinated acetylenes, resulting in the production of five-membered oxygen-containing heterocycles. For example, the Kirsch group reported that the reaction of 2-alkynyl-2-hydroxyl carbonyl compounds 50 with transition metal catalysts afforded multisubstituted 3(2H)-furanones 54 via a cascade reaction (Scheme 15).20 This transformation was induced by either PtCl2 or AuCl3, with the former giving slightly higher yields for the transformation. Activation of the alkyne moiety by either a platinum or gold catalyst allowed for heterocyclization to give the transient oxonium intermediate 52. Finally, 1,2-shift of the substituent R2 and elimination of the platinum species resulted in the formation of furanones 54. In this system, the synthetically challenging spiro furanones, in which the substituents R1 and R2 were linked, were also successfully prepared.
Another elegant example of a cationic gold-catalyzed cyclization was developed by the Maulide group. They developed the Ph3PAuCl/AgSbF6-catalyzed carbocyclization of propargyl β-keto esters 55 to give 59, in which the furan and lactone rings were constructed simultaneously (Scheme 16).21 Initial π-coordination of alkyne moiety of 55 to the cationic gold species enhanced its electrophilicity. The subsequent 5-exo-dig cyclization of the ylidic carbon with the gold-coordinated alkyne resulted in the formation of the gold–vinyl complexes 57 bearing a lactone ring. This intermediate then underwent nucleophilic attack of the acyl oxygen to release Ph2S. Finally, the furan ring was formed along with regeneration of the cationic gold catalyst (58→59). This mechanism was confirmed by exploratory density functional theory (DFT) calculations.
3. CASCADE PROCESSES INITIATED BY REARRANGEMENTS
The transition metal-promoted sigmatropic rearrangements of propargyl alcohol derivatives provided a range of different reactive intermediates, which served to construct five-membered oxygen heterocycles. In this section, the reactions are split into two categories according to the functional groups that are incorporated into the ring formation.
3.1 Participation of both the acetylene and propargylic oxygen-substituent moieties in ring formation
The reaction of diyne acylates 60 with 5 mol% of [Cu(MeCN)4][BF4] resulted in the formation of furans 65 (Scheme 17).22 The reaction was initiated by the [3,3]-sigmatropic rearrangement of 60 to allenyl esters 62 via the copper-activated electron-rich acetylene 61. This rearrangement was followed by a copper-catalyzed multistep furan formation, consisting of the following steps: 1) 1,3-acyl shift from 62 to 63; 2) the 5-exo-dig cyclization (63→64) via nucleophilic attack of the carbonyl group to the copper-coordinated acetylene; and 3) the dimerization of copper (2-furyl)carbene complexes 64 to give furans 65, which was comprised of two furan moieties, both derived from the acetylene and the acetyloxy substituent of 60.
Furthermore, the reactive intermediates 64 were suitable for subjecting to further transformations, such as hydrosilylation, hydrogermanation, oxidation, or cross coupling with a diazo compound, resulting in the formation of a range of multisubstituted furans such as those shown in Figure 4.23
In addition to C–O bond formation (see 60→62 in Scheme 17), the [3,3]-sigmatropic rearrangement of propargyl alcohol derivatives can also provide an access to C–C bond formation. In 2005, Kirsch et al. developed the transformation of propargyl vinyl ethers 66 into furans 69 using a cationic gold catalyst, generated in situ from gold and silver compounds (Scheme 18).24 The [3,3]-sigmatropic rearrangement of 66 was initiated by the π-activation of the acetylene bond with the cationic gold catalyst, followed by heterocyclization of 2,3-allenyl dione intermediates 68 to give tri- and tetrasubstituted furans 69, derived from both the propargyl and enol moieties.
The Jiang group later reported the transition metal-catalyzed one-pot cascade process, starting from propargyl alcohols 70 and electron deficient alkynes 71, in the presence of a catalytic amount of DABCO or Bu3P (Scheme 19).25 Interestingly, variation of the transition metal catalyst resulted in the formation of a range of furans bearing different substituent groups. For example, the combined catalyst of AgOAc and Ph3P in toluene converted 66, generated in situ from 70 and 71, into 69.25c However, the use of either CuI or Fe(ClO4)3·xH2O gave 2-formylfurans 73.25b,d The mechanism of the silver-catalyzed reaction was considered to be similar to that of the gold-catalyzed reaction shown in Scheme 18, whereas the copper- or iron-catalyzed reaction appeared to proceed through carbene intermediates 72, which were subsequently oxidized by oxygen to give 73.
Furthermore, the palladium-catalyzed cascade reactions, initiated by the addition of 74 to 75, provided vinyl-substituted furans 76 and 77, in which the position of the vinyl group in the products varied depending on the additives employed in the reaction. For example, the use of Bu4NF led to the formation of 4-vinyl furans 76, whereas a combination of Na2CO3 and CuCl2·2H2O gave 5-vinyl isomers 77 (Scheme 20).26 These differences arose from the different reaction paths instigated by various additives.
3.2 Participation of either the acetylene or the propargylic oxygen-substituent moiety in ring formation
The Gevorgyan group vigorously advanced the research relating to the use of a range of transition metal catalysts for the intramolecular cyclizations of propargyl esters bearing another carbonyl group.27b In the presence of 5 mol% of CuCl, 3-acylpropargyl phosphates 78 underwent a [3,3]-rearrangement of the propargyl phosphate moiety to give allenes 79, and the subsequent intramolecular addition of the carbonyl group to the allene moiety gave trisubstituted furans 80 (Scheme 21).
Similarly, the reaction of 1-benzoylpropargyl phosphate 81 with 5 mol% of AgBF4 afforded tetrasubstituted furan 83 (Scheme 22a).27 Tosylate 84 was found to spontaneously undergo the [3,3]-rearrangement to give allene 85, which was subsequently cyclized to furan 86 in the presence of 1 mol% of AgBF4 (Scheme 22b).
On a similar note, 3-acylpropargyl carboxylates 87 were converted into furans 90 upon treatment with CuCl and Et3N (Scheme 23).27 The first step in this reaction involves the 1,3-migration of the propargyl hydrogen on 87 to afford allene intermediates 88, which subsequently undergoes 1,2-acyloxy shift and heterocyclization to give furans 90.
The reaction of propargyl carboxylates 91, bearing an acyl group at the C1-position, in the presence of a silver, copper, or gold catalyst resulted in a metal-mediated 1,2-migration of the acyloxy group to generate the transient metal–carbenoids 92, which subsequently afforded tetrasubstituted furans 93 (Scheme 24).27
The reaction of propargyl diol monobenzoates with 2 mol% of Ph3PAuNTf2 was reported by Gagosz for the production of functionalized 2,5-dihydrofurans.28 A typical example involves the transformation of enantio-enriched 94 into 2,5-dihydrofuran 96 with complete retention of the chiral integrity (Scheme 25). This transformation was initiated by the [3,3]-sigmatropic rearrangement of 94 to give allene intermediate 95, which underwent heterocyclization by attack of the hydroxyl group on the gold-coordinated allene. In contrast to previously reported reactions, the hydroxyl group at the opposite propargylic position reacted ahead of the benzoyloxy group.
Zhang et al. have also studied the general reactivity of propargyl carboxylates by means of various transition metals. As a part of their ongoing research, they discovered that propargyl ester 97 bearing an indole moiety reacted with a cationic gold complex to afford the tetracyclic γ-lactone 98 bearing a 2,3-indoline-fused cyclobutane.29a The use of a platinum catalyst demonstrated nicely the difference in reactivity with variation in the metal catalyst. They observed that the substrate 97 yielded the fused γ-lactone 99, bearing a cyclopentene moiety as opposed to the cyclobutane observed using a gold catalyst (Scheme 26).29b
The mechanisms of both the gold- and platinum-catalyzed reactions of 97 begin with a [3,3]-sigmatropic rearrangement via the activation of the acetylene group by coordination to the metals. The generated allenes 100 are further activated by each catalyst to give the metal-complexed oxoniums 101. The intramolecular electrophilic cyclization at the indole C-3 position then leads to the formation of spiro intermediates 102, containing a vinyl–metal species. In the case of gold catalysis, the vinyl–gold moiety (Met = Ph3PAu+) combines with the iminium species to give 98. Conversely, in the case of platinum catalysis (Met = PtCl2), the complex undergoes a 5-exo cyclization followed by hydride shift (103→104). Elimination of the platinum species gives the fused γ-lactone 99 (Scheme 27).
4. CASCADE PROCESSES INITIATED BY PROPARGYLIC ELIMINATION
Aurrecoechea et al. reported the synthesis of multisubstituted furans 107 from epoxypropargyl esters 105, using a combination of Pd(PPh3)4 (5 mol%) and SmI2 (2 equiv) (Scheme 28).30 The reaction of 105 with SmI2 caused the reductive elimination of the acyloxy group at the propargylic position to afford enynols 106, which subsequently underwent palladium-catalyzed heterocyclization, although the yields of products 107 were by no means satisfactory. Low yields are owing to the fact that enynol intermediates 106 were generated as a mixture of Z- and E-isomers, in which only the minor Z-isomers were converted into furans 107, while the major E-isomers remained mainly unreacted due to a sluggish cyclization rate.
The Aurrecoechea group later reported that the reaction of regioisomeric epoxypropargyl esters 108 resulted in the formation of trisubstituted furans 111 (Scheme 29).31 The first step in this one-pot process involves reduction of 108 by SmI2 to generate hydroxymethyl-1,2,3-trienes 109. After the complete consumption of 108, Pd(OAc)2(PPh3)2, H2O, and AcOH are added directly to the reaction mixture. In the second step, the palladium catalyst promotes heterocyclization to give the transient intermediates 110, which are protonated with AcOH to produce 111. When aryl halides 112 or electron-withdrawing alkenes 114 are added in the second step, their coupling with intermediates 110 occurs to afford tetrasubstituted furans 113, 115, and 116 (Schemes 30a and 30b).32
Pale et al. demonstrated that nucleophiles play an important role in the cationic gold-mediated formation of furans 123 from 108a. While the reaction of 108 with Ph3PAuCl and AgSbF6 gave divinyl ketones 119 (Scheme 31a),33a the presence of nucleophiles 120, such as alcohols and thiols, furnished 123 under the same conditions (Scheme 31b).33b Although the authors described the possibility of several mechanistic pathways for the transformation, one plausible mechanism is as follows: 108a undergoes a gold-catalyzed
1,2-acyloxy migration, concomitant with the epoxide opening at the propargylic position, to afford intermediates 121, to which the addition of 120 follows. The secondary hydroxyl group present in 122 then attacks the allene moiety through assistance from coordination of the gold catalyst, and subsequent release of acetic acid gives 123.
5. OTHER TYPES OF RING FORMATION
We disclosed that a combination of (p-CF3C6H4)3PAuCl and AgOTf catalyzed the intramolecular cyclization of 124 at room temperature to afford highly substituted 3(2H)-furanones 54 (Table 3).8b This method provided good isolated yields of 54 and demonstrated excellent substrate generality. Even in the presence of a sterically demanding t-Bu substituent, the desired product was obtained in 75% yield (entry 3). The reaction was applicable to the substrate 124, bearing an alkenyl group as R3, for the direct synthesis of 5-(1-alkenyl)-3(2H)-furanones (entry 7). Such compounds have been synthesized by the strong base-mediated aldol reaction of 5-alkyl-3(2H)-furanones with aldehydes followed by dehydration, although in only modest yields and with the unsatisfactory E/Z-stereoselectivity. Our method, however, resulted in the formation of the desired product in 94% yield with retention of the olefinic configuration.
Our proposed mechanism for this transformation is shown in Scheme 32. The concomitant coordination of both alkynyl and the carbonyl groups of 124 to a cationic gold species enhances the electrophilicity of the acetylene of 125. This accelerates the subsequent Michael addition of the internal hydroxyl group, leading to the formation of the transient epoxide intermediate 127, which then cyclized through nucleophilic attack of the carbonyl oxygen to afford 54.
Another useful method for the formation of butenolides is the hetero-Pauson–Khand reaction, which is initiated by the activation of the acetylene moiety by transition metals. Recently, Adrio and Carretero developed a stereoselective synthesis for the preparation of fused bicyclic compounds exo-129 from propargyl ethers 128 in the presence of a stoichiometric amount of Mo(CO)3(DMF)3 under mild conditions (Scheme 33a).34 This method was also applied to the synthesis of (+)-dihydrocanadensolide 132, an epimer of a biologically active metabolite from Penicillium canadense (Scheme 33b).
The final reaction which we would like to discuss is a new intramolecular cyclization reported by Yoshida et al.35 The reaction involves the intramolecular cyclization of propargyl β-keto esters 133 through a Pd2(dba)3·CHCl3-catalyzed decarboxylative process to yield tetrasubstituted furans 138 (Scheme 34). In this system, the palladium catalyst allowed C–O bond cleavage at the propargyl position of 133 to generate the π-propargylpalladium enolate 134 with the concomitant release of CO2. Subsequently, 134 underwent the formal [3+2]-cyclization to give 138.
6. CONCLUSION
In this review, we have summarized the recent progress on the synthesis of five-membered oxygen heterocycles, including furans, dihydrofurans, and butenolides, starting from functionalized propargyl alcohols and their derivatives, via a number of intramolecular cyclizations. These transformations are initiated by a wide variety of transition metal-catalyzed reactions of propargyl alcohol derivatives, such as nucleophilic cyclizations, rearrangements, and eliminations. Because these types of reactions are receiving an increasing attention in terms of their evaluation for use in regioselective synthesis of highly substituted five-membered oxygen heterocycles, further investigation is expected in this field.
References
1. (a) B. A. Keay, J. M. Hopkins, and P. W. Dibble, ‘Comprehensive Heterocyclic Chemistry III’, Vol. 3, ed. by G. Jones and C. A. Ramsden, Elsevier, Amsterdam, 2008, p. 571; CrossRef (b) A. Bartoli, F. Rodier, L. Commeiras, J.-L. Parrain, and G. Chouraqui, Nat. Prod. Rep., 2011, 28, 763. CrossRef
2. (a) Z. Cui, Y. Li, Y. Ling, J. Huang, J. Cui, R. Wang, and X. Yang, Eur. J. Med. Chem., 2010, 45, 5576; CrossRef (b) T. Wakimoto, H. Kondo, H. Nii, K. Kimura, Y. Egami, Y. Oka, M. Yoshida, E. Kida, Y. Ye, S. Akahoshi, T. Asakawa, K. Matsumura, H. Ishida, H. Nukaya, K. Tsuji, T. Kan, and I. Abe, Proc. Natl. Acad. Sci. U. S. A., 2011, 108, 17533; CrossRef (c) F. Chang, S. Dutta, J. J. Becnel, A. S. Estep, and M. Mascal, J. Agric. Food Chem., 2014, 62, 476; CrossRef (d) J. Che, C.-J. Zheng, M.-X. Song, Y.-J. Bi, Y. Liu, Y.-J. Li, Y. Wu, L.-P. Sun, and H.-R. Piao, Med. Chem. Res., 2014, 23, 426; CrossRef (e) K. M. Targowska-Duda, D. Feuerbach, G. Biala, K. Jozwiak, and H. R. Arias, Neurosci. Lett., 2014, 569, 126. CrossRef
3. (a) A. Gandini, A. J. D. Silvestre, C. P. Neto, A. F. Sousa, and M. Gomes, J. Polym. Sci. Part A: Polym. Chem., 2009, 47, 295; CrossRef (b) J. A. Fernandes, Y. Morisaki, and Y. Chujo, J. Polym. Sci. Part A: Polym. Chem., 2011, 49, 3664; CrossRef (c) Z. Xu, Y. Zhao, X. Wang, and T. Lin, Chem. Commun., 2013, 49, 6755. CrossRef
4. (a) X. L. Hou, H. Y. Cheung, T. Y. Hon, P. L. Kwan, T. H. Lo, S. Y. Tong, and H. N. C. Wong, Tetrahedron, 1998, 54, 1955; CrossRef (b) N. B. Carter, A. E. Nadany, and J. B. Sweeney, J. Chem. Soc., Perkin Trans. 1, 2002, 2324; CrossRef (c) T. Graening and F. Thrun, ‘Comprehensive Heterocyclic Chemistry III’, Vol. 3, ed. by G. Jones and C. A. Ramsden, Elsevier, Amsterdam, 2008, p. 497; CrossRef (d) S. F. Kirsch, Org. Biomol. Chem., 2006, 4, 2076; CrossRef (e) H. C. Shen, Tetrahedron, 2008, 64, 3885; CrossRef (f) N. T. Patil and Y. Yamamoto, Chem. Rev., 2008, 108, 3395; CrossRef (g) T. M. Ugurchieva and V. V. Veselovsky, Russ. Chem. Rev., 2009, 78, 337. CrossRef
5. For selected reviews, see: (a) R. C. D. Brown, Angew. Chem. Int. Ed., 2005, 44, 850; CrossRef (b) A. V. Gulevich, A. S. Dudnik, N. Chernyak, and V. Gevorgyan, Chem. Rev., 2013, 113, 3084. CrossRef
6. (a) N. Marion and S. P. Nolan, Angew. Chem. Int. Ed., 2007, 46, 2750; CrossRef (b) V. Cadierno and J. Gimeno, Chem. Rev., 2009, 109, 3512; CrossRef (c) C.-H. Ding and X.-L. Hou, Chem. Rev., 2011, 111, 1914; CrossRef (d) B. Biannic and A. Aponick, Eur. J. Org. Chem., 2011, 6605; CrossRef (e) E. B. Bauer, Synthesis, 2012, 44, 1131. CrossRef
7. (a) M. Egi, Y. Yamaguchi, N. Fujiwara, and S. Akai, Org. Lett., 2008, 10, 1867; CrossRef (b) M. Egi, M. Umemura, T. Kawai, and S. Akai, Angew. Chem. Int. Ed., 2011, 50, 12197; CrossRef (c) M. Egi, T. Kawai, M. Umemura, and S. Akai, J. Org. Chem., 2012, 77, 7092. CrossRef
8. (a) M. Egi, K. Azechi, and S. Akai, Org. Lett., 2009, 11, 5002; CrossRef (b) M. Egi, K. Azechi, M. Saneto, K. Shimizu, and S. Akai, J. Org. Chem., 2010, 75, 2123; CrossRef (c) M. Egi, K. Azechi, and S. Akai, Adv. Synth. Catal., 2011, 353, 287; CrossRef (d) M. Egi, Y. Ota, Y. Nishimura, K. Shimizu, K. Azechi, and S. Akai, Org. Lett., 2013, 15, 4150. CrossRef
9. Y. Wakabayashi, Y. Fukuda, H. Shiragami, K. Utimoto, and H. Nozaki, Tetrahedron, 1985, 41, 3655. CrossRef
10. M. Sakai, M. Sasaki, K. Tanino, and M. Miyashita, Tetrahedron Lett., 2002, 43, 1705. CrossRef
11. S. J. Hayes, D. W. Knight, M. D. Menzies, M. O’Halloran, and W.-F. Tan, Tetrahedron Lett., 2007, 48, 7709. CrossRef
12. A. Aponick, C.-Y. Li, J. Malinge, and E. F. Marques, Org. Lett., 2009, 11, 4624. CrossRef
13. (a) F. E. McDonald, C. B. Connolly, M. M. Gleason, T. B. Towne, and K. D. Treiber, J. Org. Chem., 1993, 58, 6952; CrossRef (b) F. E. McDonald and M. M. Gleason, J. Am. Chem. Soc., 1996, 118, 6648. CrossRef
14. Y. Yada, Y. Miyake, and Y. Nishibayashi, Organometallics, 2008, 27, 3614. CrossRef
15. (a) B. Gabriele, L. Veltri, R. Mancuso, P. Plastina, G. Salerno, and M. Costa, Tetrahedron Lett., 2010, 51, 1663; CrossRef (b) B. Gabriele, R. Mancuso, V. Maltese, L. Veltri, and G. Salerno, J. Org. Chem., 2012, 77, 8657. CrossRef
16. R. Spina, E. Colacino, J. Martinez, and F. Lamaty, Chem. Eur. J., 2013, 19, 3817. CrossRef
17. (a) A. Blanc, K. Tenbrink, J.-M. Weibel, and P. Pale, J. Org. Chem., 2009, 74, 4360; CrossRef (b) A. Blanc, K. Tenbrink, J.-M. Weibel, and P. Pale, J. Org. Chem., 2009, 74, 5342. CrossRef
18. (a) F. E. McDonald and C. C. Schultz, J. Am. Chem. Soc., 1994, 116, 9363; CrossRef (b) C.-Y. Lo, H. Guo, J.-J. Lian, F.-M. Shen, and R.-S. Liu, J. Org. Chem., 2002, 67, 3930; CrossRef (c) A. S. K. Hashmi and P. Sinha, Adv. Synth. Catal., 2004, 346, 432; CrossRef (d) M. Yoshida, M. Al-Amin, and K. Shishido, Synthesis, 2009, 2454. CrossRef
19. X.-Z. Shu, X.-Y. Liu, H.-Q. Xiao, K.-G. Ji, L.-N. Guo, C.-Z. Qi, and Y.-M. Liang, Adv. Synth. Catal., 2007, 349, 2493. CrossRef
20. (a) S. F. Kirsch, J. T. Binder, C. Liébert, and H. Menz, Angew. Chem. Int. Ed., 2006, 45, 5878; CrossRef (b) J. T. Binder, B. Crone, S. F. Kirsch, C. Liébert, and H. Menz, Eur. J. Org. Chem., 2007, 1636. CrossRef
21. X. Huang, B. Peng, M. Luparia, L. F. R. Gomes, L. F. Veiros, and N. Maulide, Angew. Chem. Int. Ed., 2012, 51, 8886. CrossRef
22. J. Barluenga, L. Riesgo, R. Vicente, L. A. López, and M. Tomás, J. Am. Chem. Soc., 2007, 129, 7772. CrossRef
23. J. Barluenga, L. Riesgo, R. Vicente, L. A. López, and M. Tomás, J. Am. Chem. Soc., 2008, 130, 13528. CrossRef
24. M. H. Suhre, M. Reif, and S. F. Kirsch, Org. Lett., 2005, 7, 3925. CrossRef
25. For a review, see: (a) H. Cao, H. Jiang, and H. Huang, Synthesis, 2011, 1019; CrossRef For some examples, see: (b) H. Cao, H. Jiang, W. Yao, and X. Liu, Org. Lett., 2009, 11, 1931; CrossRef (c) H. Cao, H. Jiang, R. Mai, S. Zhu, and C. Qi, Adv. Synth. Catal., 2010, 352, 143; CrossRef (d) H. Jiang, W. Yao, H. Cao, H. Huang, and D. Cao, J. Org. Chem., 2010, 75, 5347. CrossRef
26. H. Huang, H. Jiang, H. Cao, J. Zhao, and D. Shi, Tetrahedron, 2012, 68, 3135. CrossRef
27. (a) A. W. Sromek, A. V. Kel`in, and V. Gevorgyan, Angew. Chem. Int. Ed., 2004, 43, 2280; CrossRef (b) T. Schwier, A. W. Sromek, D. M. L. Yap, D. Chernyak, and V. Gevorgyan, J. Am. Chem. Soc., 2007, 129, 9868. CrossRef
28. A. Buzas, F. Istrate, and F. Gagosz, Org. Lett., 2006, 8, 1957. CrossRef
29. (a) L. Zhang, J. Am. Chem. Soc., 2005, 127, 16804; CrossRef (b) G. Zhang, V. J. Catalano, and L. Zhang, J. Am. Chem. Soc., 2007, 129, 11358. CrossRef
30. J. M. Aurrecoechea and M. Solay-Ispizua, Heterocycles, 1994, 37, 223. CrossRef
31. J. M. Aurrecoechea, E. Pérez, and M. Solay, J. Org. Chem., 2001, 66, 564. CrossRef
32. (a) J. M. Aurrecoechea and E. Pérez, Tetrahedron, 2004, 60, 4139; CrossRef (b) J. M. Aurrecoechea, A. Durana, and E. Pérez, J. Org. Chem., 2008, 73, 3650. CrossRef
33. (a) M.-C. Cordonnier, A. Blanc, and P. Pale, Org. Lett., 2008, 10, 1569; CrossRef (b) A. Blanc, A. Alix, J.-M. Weibel, and P. Pale, Eur. J. Org. Chem., 2010, 1644. CrossRef
34. J. Adrio and J. C. Carretero, J. Am. Chem. Soc., 2007, 129, 778. CrossRef
35. M. Yoshida, S. Ohno, and K. Shishido, Chem. Eur. J., 2012, 18, 1604. CrossRef