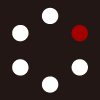
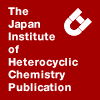
HETEROCYCLES
An International Journal for Reviews and Communications in Heterocyclic ChemistryWeb Edition ISSN: 1881-0942
Published online by The Japan Institute of Heterocyclic Chemistry
e-Journal
Full Text HTML
Received, 10th March, 2015, Accepted, 14th April, 2015, Published online, 27th April, 2015.
DOI: 10.3987/COM-15-13208
■ Synthesis of 1,5-Dioxaspiro[3.4]octane through Bromocation-Induced Cascade Cyclization
Atsuo Nakazaki, Yoshiki Nakane, Yuki Ishikawa, and Toshio Nishikawa*
Laboratory of Organic Chemistry, Graduate School of Bioagricultural Sciences, Nagoya University, Furo-cho, Chikusa, Nagoya, Aichi 464-8601, Japan
Abstract
A new approach to the synthesis of 1,5-dioxaspiro[3.4]octane, spiro-oxetane having acetal moiety, via the bromocation-induced cyclization is described. This reaction underwent to furnish spiro-oxetane in one-pot manner with a high degree of diastereoselectivity.Much attention has been centered on bicyclic oxetane containing an acetal carbon in the cyclic framework because of unique structural features and their biological activities (Figure 1).1 A representative example is thromboxane A2, which is known to be a platelet aggregation factor and extremely labile substance (T1/2 = 32 sec in aqueous Krebs medium at pH 7.4).2-4 Owing to the highly strained nature of oxetane nucleus, synthetic approaches are limited.1 In particular, bicyclic oxetane containing an acetal should be constructed through kinetically controlled reactions in order to avoid decomposition of the formed oxetane which potentially bears thermodynamic instability originated from acetal moiety. Bicyclic oxetane A in Figure 1 is available via Williamson ether synthesis4,5 or Mitsunobu reaction.3 In contrast, bicyclic oxetane B6 and spirocyclic oxetane C (1,5-dioxaspiro[3.4]octane)7 are known to be constructed by utilizing [2+2] cycloaddition of carbonyl compound and alkene.
In this communication, we describe a new approach to the synthesis of 1,5-dioxaspiro[3.4]octane via the cascade bromocyclization (Scheme 1).8,9 Although used unsymmetrical diol D would mechanistically provide two 1,5-dioxaspiro[3.4]octanes C' and C'' through bromocation-induced 5-endo-dig followed by 4-exo-trig cyclizations,10 only spiro-oxetane C' was observed.
In the course of our synthetic study on spirocyclic guanidine alkaloid,9 we have unexpectedly found the formation of a spirocyclic oxetane under the cascade bromocyclization conditions developed in this group8 (Scheme 2). Thus, diol 111 was subjected to pyridinium tribromide (PyHBr3) in the presence of
K2CO3 in a biphasic solvent system (CH2Cl2 and H2O, 1:1) to furnish 1,5-dioxaspiro[3.4]octane 212 in 27% yield as a single diastereomer instead of the expected spiro-hemiaminal 3. Interestingly, the obtained spiro-oxetane 2 was found to be stable enough to be isolated by neutral silica gel column chromatography probably due to the effect of geminal dibromomethylene by destabilizing oxonium ion.3,4 In this process, guanidino-diol 1 underwent bromocation-induced cyclization to generate a cyclic enol ether, which was further reacted with bromocation to form oxonium ion intermediate. And then the oxonium ion would be intercepted by an internal hydroxy group to furnish spiro-acetal 2 having an oxetane ring.13 No observation of the expected spiro-hemiaminal 3 would be attributed to sluggish rate of the nucleophilic addition of a nitrogen atom of the (biscarbamoyl)guanidino group. To the best of our knowledge, this is the first example for the construction of a 1,5-dioxaspiro[3.4]octane scaffold through electrophilic cyclization.
We next investigate the related reaction by utilizing simplified diol 4 without guanidine functionality in order to clarify a generality of the reaction (Scheme 3). Thus, the cascade bromocyclization using diol 414 took place to afford spiro-oxetane 5 in 24% yield along with hemiketal 6 in 28% yield; spiro-oxetane 7 was not detected. Both compounds were obtained as a single diastereomer. Relative stereochemistry of the resulting oxetane 5 was confirmed by NOESY analysis of monobromo compound 8 via reductive debromination of 5.15 The stereogenic center of the methine proton adjacent to the bromo substituent in 8 could not be determined because of almost the same distance between both methine protons in the most stable conformer calculated by molecular mechanics. Spirocyclic monobromo oxetane 816 was found to
be more labile than the corresponding dibromo oxetane 5 because of less electronegative nature of the monobromo-substituted carbon.
A plausible reaction mechanism of the cascade bromocyclization of diol 4 is shown in Scheme 4. Bromocation activates alkyne of 4 providing bromonium ion intermediate 9, in which less hindered hydroxy group cyclizes through path a in a 5-endo-dig manner, leading to dihydrofuran 10. The second activation of 10 by bromocation with concomitant cyclization again furnishes spiro-oxetane 5. The side-chain substituent might promote the rate of cyclization owing to compressing the angle of its substituted carbon.17 In contrast, path b affords dihydrofuran 11, which would be activated by bromocation to generate oxonium ion intermediate 12. At this stage, 4-exo-trig cyclization of the remaining hydroxy group would be retarded probably due to no substituent effect compressing the angle at that carbon, and intermolecular attack of water proceeds to form hemiketal 6. Formation of 7 and its subsequent hydrolysis might also be possible for explaining production of 6.
Stereochemical outcome of spiro-oxetane 5 indicates that the second cyclization would proceed through sterically less-hindered conformer 13, instead of the conformer 13' involving a steric repulsion between gem-dibromomethylene moiety and phenylethyl group, leading to 5 as a sole diastereomer (Scheme 5).
In conclusion, we described a unique access to the synthesis of spirocyclic oxetane acetal under the bromocation-induced cyclization conditions. The feature of this method is that the cyclization underwent to furnish spiro-oxetane in one-pot manner with a high degree of diastereoselectivity. A geminal dibromomethylene group might play an important role for stabilizing substituent of the acetal moiety.
ACKNOWLEDGEMENTS and notes
This work was financially supported by a Grant-in-Aid for Scientific Research on Innovative Areas “Chemical Biology of Natural Products” and a Grant-in-Aid for Young Scientists (B) from The Ministry of Education, Culture, Sports, Science and Technology, Japan; Grant for Basic Science Research Projects from The Sumitomo Foundation; and the Daiichi-Sankyo Foundation of Life Science.
References
1. For reviews of oxetanes, see: (a) E. M. Carreira and T. C. Fessard, Chem. Rev., 2014, 114, 8257; CrossRef (b) J. A. Burkhard, G. Wuitschik, M. Rogers-Evans, K. Müller, and E. M. Carreira, Angew. Chem. Int. Ed., 2010, 49, 9052. CrossRef
2. M. Hamberg, J. Svensson, and B. Samuelsson, Proc. Natl. Acad. Sci. U.S.A., 1975, 72, 2994. CrossRef
3. (a) S. S. Bhagwat, P. R. Hamann, and W. C. Still, J. Am. Chem. Soc., 1985, 107, 6372; CrossRef (b) S. S. Bhagwat, P. R. Hamann, and W. C. Still, Tetrahedron Lett., 1985, 26, 1955; CrossRef (c) S. S. Bhagwat, P. R. Hamann, W. C. Still, S. Bunting, and F. A. Fitzpatrick, Nature, 1985, 315, 511. CrossRef
4. J. Fried, E. A. Hallinan, and M. J. Szwedo, Jr., J. Am. Chem. Soc., 1984, 106, 3871.
5. (a) F. A. Khan, G. H. M. Rao, R. Satapathy, and K. Parasuraman, Org. Lett., 2007, 9, 1581; CrossRef (b) H. Ito, R. Eby, S. Kramer, and C. Schuerch, Carbohydr. Res., 1980, 86, 193. CrossRef
6. A. G. Griesbeck, S. Buhr, M. Fiege, H. Schmickler, and J. Lex, J. Org. Chem., 1998, 63, 3847, and references therein. CrossRef
7. (a) J. Brunckova and D. Crich, Tetrahedron, 1995, 51, 11945; CrossRef (b) B. Pandey, R. S. Reddy, and P. Kumar. J. Chem. Soc., Chem. Commun., 1993, 870; CrossRef (c) W. Adam, U. Kliem, and V. Lucchini, Liebigs Ann. Chem., 1988, 869; (d) K. Maruyama, T. Ogawa, and Y. Kubo, Chem. Lett., 1980, 343; CrossRef (e) Y. Araki, K. Senna, K. Matsuura, and Y. Ishido, Carbohydr. Res., 1978, 65, 159; CrossRef (f) G. Kaupp and M. Stark, Angew. Chem., Int. Ed. Engl., 1978, 17, 758; CrossRef (g) S. Farid and S. E. Shealer, J. Chem. Soc., Chem. Commun., 1973, 296. CrossRef
8. (a) Y. Sawayama and T. Nishikawa, J. Synth. Org. Chem. Jpn., 2012, 70, 1178; CrossRef (b) Y. Sawayama and T. Nishikawa, Angew. Chem. Int. Ed., 2011, 50, 7176; CrossRef (c) Y. Sawayama and T. Nishikawa, Synlett, 2011, 651.
9. A. Nakazaki, Y. Ishikawa, Y. Sawayama, M. Yotsu-Yamashita, and T. Nishikawa, Org. Biomol. Chem., 2014, 12, 53. CrossRef
10. For examples of the formation of oxetane through bromocation-induced cyclization, see: (a) M.-Y. Chang, C.-Y. Tsai, and M.-H. Wu, Tetrahedron, 2013, 69, 6364; CrossRef (b)S. Albert, S. Robin, and G. Rousseau, Tetrahedron Lett., 2001, 42, 2477; CrossRef (c) F. Homsi and G. Rousseau, J. Org. Chem., 1999, 64, 81; CrossRef (d) M. Rofoo, M.-C. Roux, and G. Rousseau, Tetrahedron Lett., 2001, 42, 2481; CrossRef (e) E. Ehlinger and P. Magnus, J. Am. Chem. Soc., 1980, 102, 5004. CrossRef
11. Cyclization precursor 1 was synthesized by the similar way as previously reported method.9 See Supporting Information for details.
12. Relative stereochemistry of 2 was not determined.
13. An alternative reaction mechanism leading to 2 can be imagined involving bromocation-induced 4-exo-dig cyclization of guanidino-diol 1 followed by 5-exo-trig cyclization. However this reaction would be suppressed because of instability of an oxonium ion intermediate having oxetane scaffold which would be generated after the first cyclization.
14. Cyclization precursor 4 was synthesized from hydrocinnamaldehyde in four steps. See Supporting Information for details.
15. Both recovered oxetane 5 and totally reduced product were not observed in this reaction.
16. Monobromide 8 was found to be stable during the separation using alumina or neutralized silica gel (Silica gel 60N, Kanto) with 10% of i-Pr2NH as a co-eluent, however decomposition of 8 was observed on TLC plate (Silica gel 60, Merck).
17. For review of Thorpe-Ingold effect as a related effect, see: M. E. Jung and G. Piizzi, Chem. Rev., 2005, 105, 1735 CrossRef