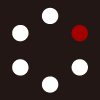
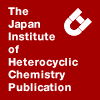
HETEROCYCLES
An International Journal for Reviews and Communications in Heterocyclic ChemistryWeb Edition ISSN: 1881-0942
Published online by The Japan Institute of Heterocyclic Chemistry
e-Journal
Full Text HTML
Received, 25th September, 2015, Accepted, 10th December, 2015, Published online, 18th December, 2015.
DOI: 10.3987/REV-15-827
■ Nanostrucure Formation Based on the Functionalized Side Chains in Liquid-Crystalline Heteroaromatic Compounds
Atsushi Seki and Masahiro Funahashi*
Deparment of Advanced Materials Science, Faculty of Engineering, Kagawa University, 2217-20 Hayashi-cho, Takamatsu, Kagawa 761-0396, Japan
Abstract
Heteroaromatic skeletons are important functional units for optic and electronic materials. For the construction of novel functional soft matter, the self-assembled system utilizing the heteroaromatic molecules bearing various substituents provide an effective approach. Recently, a lot of liquid crystals and fibrous aggregates with electronic functions have been developed. Among them, liquid-crystalline (LC) semiconductors are well-known examples. LC semiconductors are hopeful candidates which cope with both carrier transport property and solubility. Although the optimization of the chemical structures of the central aromatic core has been mainly considered in the past study, side chains also play a key role to form the well-defined nanosegregated superstructures. The structural optimization of the side chains as well as the rigid cores contributes to the developments of the “soft matter electronics”. Herein, we will review the recent research trend on functional π-conjugated liquid crystals focusing on the flexible side chains. Furthermore, we will describe the importance of the nanosegregation for the design of soft materials having electronic functions.1. INTRODUCTION
Heteroaromatic compounds possessing heteroatoms such as nitrogen, sulfur and oxygen show quite different features from those of aromatic hydrocarbons. For instance, the introduction of heteroatoms to the aromatic backbones affects their reactivities, redox activities, metal coordination abilities and hydrogen bonding properties. Heteroaromatic moieties are significant parts in organic functional materials and numerous studies have been performed for aiming at the application to functional dyes, optical and electronic materials based on unique heteroaromatic cycles.1
On the other hand, soft matter represented by block copolymers, physical gels consisting of fibrous aggregates and liquid crystals are characterized by self-assembled supramolecular structures of functional moieties. Molecules functionalized with heteroaromatic cycles, amide groups, alkyl chains etc. form the various supramolecular self-assemblies depending on the volume fractions of the incompatible units and intermolecular interactions. Figure 1 demonstrates a schematic illustration of supramolecular structures constructed by the molecules bearing incompatible functional moieties. In general, rod-like molecules form layer structures while fan-shaped molecules assemble into cylindrical structures. In the case of the LC molecules bearing incompatible side chains with a large difference of the free volumes, a large curvature should be generated, so that the layer or cylindrical structures are disturbed and gyroid or spherical structures are formed. The self-assembled systems provide an effective methodology for the construction of the novel functional materials based on bottom-up approach.2-4
These LC molecules to produce self-assembled supramolecular structures are composed of several incompatible segments. In general, LC molecules have flexible chains and a rigid core. Rigid cores are usually comprised of aromatic moieties and determine the optical and electronic property of the supramolecular assemblies. In contrast, flexible units are associated with the softness and flexibility of the assemblies. Dynamic ordered structures of LC phases are originated from the thermal motion of the flexible units. The freedom of the molecular motion is strongly associated with one of the most significant property of LC materials, the formation of large-area homogeneous thin films.
LC semiconductors exhibit good carrier transport properties comparable to organic polycrystalline semiconductors and conjugated polymers. From the viewpoint of the carrier transport property, a single-crystal structure in which π-conjugated molecules are stacked closely in a crystal lattice is most favorable because intermolecular π-orbital overlaps are maximized and structural disorder to inhibit charge carrier movement is minimized. However, the single-crystalline materials generally have a difficulty in the preparation of the thin film with the low defect density. Polycrystalline thin films can be produced by a vacuum process. However, their carrier transport properties are inferior to single-crystalline materials, due to the domain boundaries, which are inconsistency of the molecular orientation and are regarded as a major problem on the carrier transport property. In contrast, LC semiconductors are less affected by the domain boundaries due to their dynamic ordered structures.
In addition, LC materials have good solubility in conventional organic solvents, due to the thermal motion of the flexible side chains. In the processability, crystalline materials are unfavorable because of their poor solubility and the difficulty in the preparation of homogeneous thin films. In contrast, LC semiconductors provide the good solubility and homogeneous thin film formation property, and are suitable for device production with solution processes including a printing technology.
Up to now, the design of aromatic cores has been studied mainly for LC semiconductors. However, the roles of side chains are not negligible. The side chains of LC molecules not only disturb the molecular aggregation by their thermal motion, but also promote the formation of supramolecular assemblies through nanosegregation. Thus, the optimization of the chemical structures of the flexible side chain units as well as the rigid cores contributes to the developments of the novel multifunctional materials. Especially, the organic electronic materials based on the concept of nanosegregation will contribute to the development of the “soft matter electronics”.4d
In this highlight paper, we will focus on the LC semiconductors among the various functional liquid crystals. We will describe the novel molecular designs for them based on the flexible side chains, but not conventional aromatic cores. We will review the recent research trend on functional π-conjugated liquid crystals and emphasize the significance of the nanosegregation for the design of soft materials having electronic functions.
2. CLASSICAL LC SEMICONDUCTORS
2-1. LC SEMICONDUCTORS BASED ON AROMATIC HYDROCARBONS
Chemical structures of representative LC semiconductors are shown in Figure 2. In the aromatic hydrocarbons, triphenylene, phenyl naphthalene and perylene tetracarboxylic acid bisimide (PTCBI) are typical mesogens for LC semiconductors. Haarer’s and Boden’s groups reported LC semiconductors based on the triphenylene core with high carrier mobilities in their LC phases.5a,b Disk-like shaped LC triphenylene derivatives generally form columnar structures. 2,3,6,7,10,11-Hexahexyloxytriphenylene (1), and 2,3,6,7,10,11-hexahexylthiotriphenylene (2) are well-known examples. The triphenylene cores stack and form one-dimensional conduction paths of electronic charge carriers in their columnar LC phases. The triphenylene cores are an electron-rich aromatic unit and favorable for hole transport. The hole mobilities of these triphenylene-based LC were on the order of 10-3 - 10-2 cm2 V-1 s-1 determined by time-of-flight (TOF) method.5a,b,g-i
In addition to columnar phases consisting of disk-like LC molecules with extended π-conjugated systems, efficient carrier transport was observed in smectic phases consisting of the LC hydrocarbons having relatively small π-conjugated systems. The phenyl naphthalene derivatives (3) bearing simple alkyl chains exhibit several smectic LC phases and the hole and electron mobilities in their ordered smectic phases reach the order of 10-2 cm2 V-1 s-1.6 The PTCBI is well-known as a representative mesogenic core which shows the electron transporting property.7,8 The PTCBI core has an electron-deficient nature because of four electron-withdrawing carbonyl groups. Most of LC PTCBI derivatives exhibit the columnar phase.7d-f,9 Besides, LC PTCBI derivatives with the asymmetrical side chains or long alkyl chains form the lamellar LC structures.7a,g Sudhölter and coworkers reported a LC PTCBI derivative bearing two octadecyl chains, which form a smectic phase. The electron mobility in the LC phase was evaluated by the pulse-radiolysis time resolved microwave conductivity (PR-TRMC) method and the electron mobility reaches the order of 10-1 cm2 V-1 s-1.7a
It is noted that the carrier mobility estimated by PR-TRMC method is higher than the value estimated by a TOF method. A carrier mobility obtained by the PR-TRMC method is a band mobility, which corresponds to the rate of intermolecular charge transfer without a carrier trapping process. The value determined by the TOF technique is a drift mobility, which involves detrapping processes of charge carriers from localized states formed by impurities and defects.
2-2. LC SEMICONDUCTORS BASED ON HETEROAROMATIC MOIETIES
Not only the aromatic hydrocarbons, but heteroaromatic LC semiconductors also have been reported.10 Ohta and coworkers synthesized symmetrical and asymmetrical phthalocyanine derivatives (6, 7) which formed the columnar LC structures. They evaluated their carrier transport properties by TOF or PR-TRMC methods.5e,10c,d
Swarts and Cook’s group also synthesized LC metal-free phthalocyanines.10e Cook, Ozaki and coworkers developed a LC phthalocyanine derivative (8) octa-substituted at non-peripheral positions. They evaluated the drift carrier mobility in the crystalline and hexagonal disordered columnar phases of the phthalocyanine 8, which increases to the order of 10-1 cm2 V-1 s-1.10f In addition, they fabricated the bulk heterojunction organic photovoltaic cells using the LC phtharocyanin derivative 8 as a hole-transport material.10g The LC zinc porphyrin oligomer (9) was synthesized by Aida’s group. The metalloporphirin 9 exhibited rectangular columnar phases in which band mobilities were on the order of 10-2 cm2 V-1 s-1.10h Würthner et al. synthesized an asymmetrical zinc chlorophyll derivative (10), of which carrier mobility evaluated by the PR-TRMC method were on the order of 10-2 cm2 V-1 s-1 in the columnar LC phase.10i Shimizu and coworkers developed a LC dithienonaphthalene (DTN) derivative (11) and fabricated the high performance thin film transistors whose active layer composed of the LC DTN.10j Funahashi, Hanna and coworkers synthesized phenylbenzothiazole derivatives (12) which exhibited smectic LC phases. Ambipolar carrier transports were observed in their smectic LC phases. The hole and electron mobilities were of the order of 10-3 cm2 V-1 s-1.6,10k-l
In this way, the chemical structure of the central aromatic core has an impact on the self-assembled structure, influencing on carrier transport property. The closed packing of the π-conjugated moieties is required for the efficient carrier transport and high carrier mobilities have been observed in ordered LC phases. LC compounds bearing heteroaromatic cores exhibit redox activity,11 mechanical stimuli-responsiveness12 as well as carrier transport property.5,6 Especially, oligothiophene frameworks are considered as a substructure of polythiophenes, which are typical π-conjugated polymers. Based on the idea that organization of the oligothiophene cores in LC phases should enhance the electronic properties, LC oligothiophene have been studied for the alternative materials.4b,13 The thiophene rings prefer all-trans (transoid) conformation to all-cis (cisoid) or twisted conformations generally. As the expanding the π-conjugated planes with increasing the number of thiophene rings, the planarity of the aromatics are maintained. The extended π-conjugated system is preferable for the efficient charge carrier transport, leading to the bathochromic shift of the absorption maxima. However, the melting points of the compounds are remarkably raised and their solubilities are reduced due to the enhanced self-association of the extended π-conjugated plane.
Low solubility of the extended π-conjugated materials causes difficulties in the synthetic and purification processes. For the fabrication of practical electronic devices using wet processes represented by a printing technique, π-conjugated materials with high solubilities are indispensable. One method to increase their solubilities is the introduction of the linear alkyl chains to the heteroaromatic cores. Conventional LC molecules consist of aromatic cores and alkyl chains, and exhibit good solubility in organic solvents.
A lot of LC oligothiophene derivatives have been reported up to the present time.14 McCulloch et al. reported that the quaterthiophene derivatives (13) bearing the polymerizable acrylate groups in the terminal of the side-chains exhibit the LC properties. In the report, they prepared the field-effect transistors (FETs) with using the LC qurterthiophenes as the active layers and demonstrated the performances of the devices.14a Garnier and Horowitz et al. reported that 5,5'''-dihexyl-2,2':5', 2'':5'',2'''-quarterthiophene (DH4T) shows a smectic phase, indicating the formation of the layer structure at an elevated temperature. They also demonstrated the performance of the FETs based on the DH4T and showed that the field-effect mobility reached on the order of 10-2 cm2 V-1 s-1.14c Although various symmetrically alkylated quaterthiophene (14) and terthiophene (15) derivatives have been synthesized,14d,15 the melting points of these compounds are generally above 100 ºC and room-temperature LC oligothiophenes are quite limited.
Funahashi et al. have synthesized room-temperature oligothiophene liquid crystals (16, 17) bearing the normal linear alkyl chains.16 Especially, 3-TTPPh-5 (18) shows the ordered smectic phase in a wide temperature range including room temperature. We have observed that high hole mobility in the ordered smectic phase of 3-TTPPh-5 exceeding 10-1 cm2 V-1 s-1. We have explained that the hole transport in the LC system of 3-TTPPh-5 is based on the band-like conduction mechanism by the observation of temperature- and field-independent mobility over wide temperature range.16c Using this compound, field-effect transistors were produced by a spin-coating method. When the transistors were fabricated on polymer films, their performance was retained under the application of the strain around 10%.
Heteroaromatic LC semiconductors with heavy chalcogens have an advantage over aromatic hydrocarbon-based LC semiconductors because they have relatively large van der Waals’ radii. Increase in the overlaps between the π-molecular orbitals enhances the carrier transport. In fact, high carrier mobilities comparable to those of amorphous silicon have been achieved in the crystal phase of heteroaromatic compounds.
Recently, organic crystalline semiconductors based on thienothiophene units have been reported. The representative crystalline semiconductors based on sulfur containing aromatics are shown in Figure 3.17-20 Takimiya’s group reported that the preparation of the high performance organic field-effect-transistors using the [1]benzothieno[3,2-b][1]benzothiophene (BTBT) derivatives for crystalline active layer.17 The high carrier mobility in this system derived from the large transfer integral which is affected by the large van der Waals’ radius of sulfur atoms on the BTBT core and the Sulfur-Sulfur interaction.
Bao’s and Tsukagoshi’s groups reported that the achievements of high field-effect mobiliies on the order of 101 cm2 V-1 s-1 by controlling the molecular orientations with 2.7-dioctyl[1]benzothieno[3,2-b]-[1]benzothiophene (C8-BTBT) and the analogues (19) for active layers.18 Dinaphtho[2, 3-b:2’, 3’-f]thieno[3, 2-b]thiophene derivatives (20) also have good field-effect mobilities up to the order of 101 cm2 V-1 s-1.19 In addition, Yasuda and Adachi’s group recently reported fast carrier transport in the crystal phases of sulfur-containing aromatics (21-23) expanded the π-electron system of the dithienothiophene (DTT) skeleton utilizing the S-S contact.20 Thus, it is significant to optimize the intermolecular interactions which directly contribute to the self-assembled structures of low molecular-weight heteroaromatic compounds for the carrier transport property.
Single-crystalline materials are the most suitable for high performance devices at the sacrifice of their processability. In polycrystalline materials, the grain boundaries are an obstacle to efficient carrier transport. In contrast, LC semiconductors have a big advantage over crystalline materials in the film-forming property and processability.
In the conventional molecular design of LC semiconductors, flexible long alkyl chains are introduced to the central extended π-conjugated aromatic core. It is reported that C8-BTBT and the analogous compounds Cn-BTBT (n = 6, 10, 12) form smectic mesophases.21 In the C8-BTBT system, liquid-crystallinity of the C8-BTBT improves the solubility in the organic solvents and the molecular orientation. The DTT derivatives (21) also form smectic phases20a and the same effect should contribute to their processability. The LC systems can be considered for the construction strategy on the low-cost and high-performance electronic devices. Iino, Hanna and coworkers ascertained the effect of the LC property to the device performance. They fabricated thin film transistors using C10-BTBT, whose active layers prepared by a spin-coating technique in different temperatures at crystalline and LC phases. The device based on polycrystalline thin films spin-coated in the LC phase exhibited superior performance to the devices using the active layers spin-coated at room temperature.22 Gomez and coworkers demonstrated the optimization of the quenching temperature from melting state to crystalline phase on the C8-BTBT system. They obtained the thin film with suitable morphology for field-effect transistors by controlling of crystallization.23
As shown in Figure 4, BTBT-based LC compounds were synthesized aiming for the improvement of the device performance by structural reorganization effect in the LC system.24 Iino, Hanna and coworkers synthesized a thienyl substituted BTBT derivative (24), which exhibits smectic A and smectic E phases. They fabricated thin film transistors whose active layer was composed of the thienyl-BTBT derivative. The active layers were prepared by the spin-coating technique in the LC temperature range. They also studied on the thermal treatment effect upon the film morphology and the device performance.18a A phenyl substituted BTBT derivative (25), which exhibits smectic A and smectic E phases, was also synthesized by the same group. The device performance of the thin film transistors based on the phenyl-BTBT derivative were evaluated in wide temperature range including the LC phase temperature.24b Hasegawa et al. synthesized analogous phenyl-BTBT derivatives. They studied on the influence of the alkyl chain length upon the solubility and thermal property.24c Méry, Shimizu and coworkers synthesized a dialkenyl substituted BTBT derivative (26), which forms the lamella-columnar LC structure. They evaluated the carrier transport property by time-of-flight technique. The drift mobility in the LC phase of the compound was on the order of 10-2 cm2 V-1 s-1.24d Choi’s group synthesized thienylethenyl-BTBT derivatives (27), which exhibit smectic phases. They fabricated thin film transistors based on the thienylethenyl-BTBT derivatives.24e
On the other hand, the various aromatic systems behave as the rigid core. The aromatic rings are also electronically and spectroscopically active units due to the π-electrons. From these characteristic properties of aromatic rings, the aromatic cores have been mainly focused upon for the conventional molecular design of the functional soft materials. So far, the expansion and modification of π-conjugated moiety have been studied for the tuning of HOMO and LUMO energy levels and/or the intramolecular charge-transfer. As a result of these studies, the tuning of the redox activities and absorption properties as well as the enhancement of the carrier mobilities have been achieved.10a,10b,25 In these molecular designs, not only the aromatic hydrocarbons but also heterocycles and fused aromatic rings are used for a central core.26
3. SELF-ORGANIZATION WITH POLAR SIDE CHAINS OR POLAR UNITS
3-1. POLAR SIDE CHAINS
Conventional LC molecules consist of rigid aromatic cores and flexible side chains. The side chains weaken the strong interaction between the aromatic cores by their thermal motion to form LC phases. In conventional molecular designs of the LC compounds, non-polar alkyl chains are usually used as flexible units.
The thermodynamic stability of the LC phases has generally explained by a few models based on the classical Onsager hard-rod, Maier-Saupe (MS), and Gay-Bern (GB) models.27 All these models assume simple rigid molecules with a cylindrical symmetry and single intermolecular interaction. They are not proper for the LC molecules consisting of a few incompatible parts causing a competition of plural intermolecular interactions.
Some characteristic unconventional LC systems, which are not possible to be explained by the classical theoretical models, have recently reported.28 For the explanation of these unconventional LC systems, the concept of nanosegregation has been proposed.29 This model describes that the various LC structures are formed by the nanosegregation between the incompatible segments (e.g. polar and non-polar units) of the LC molecules. In the molecules having both the polar ionic unit and non-polar alkyl chains, the incompatible units are independently assembled to form the nanosegregated structures. We focus on the structures of side chains of the LC molecules and describe the LC material design based on nanosegregation.
The LC systems based on the polar side chains such as oligo(ethylene oxide) units,30 propylene oxide units,31 fluoroalkyl chains32 and perfluoroalkyl chains have been reported.33 These polar side-chains having larger dipole moments compared to the non-polar aliphatic and/or aromatic rings. Therefore, the side chains are immiscible with aromatic cores and aliphatic side chains, resulting in microscopic segregation on the order of nm scale. It has recently been pointed out that the nanosegregation strongly contributes to the stabilization of the LC phases.34
Figure 5 exhibits molecular structures of π-conjugated LC’s bearing polar side chains. Tschierske et al. reported that oligothiophene derivatives (28) bearing glycerol groups and lateral alkyl chains formed the hexagonal and tetragonal columnar superstructures.35 Schenning and Meijer et al. synthesized sexithiophene derivatives (29) functionalized by oligo(ethylene oxide) chains. These compounds formed the smectic layer structures associated with coiled aggregates. In the self-assembled coils, π-conjugated
rigid cores and flexible polar side chains were separately assembled.36 Gregg et al. synthesized LC PTCBI derivatives (30, 31) bearing the linear or branched oligo(ethylene oxide) chains. These compounds formed interdigitated or tilted lamellar structures. Moreover, they also evaluated the electronic properties of the PTCBI derivatives.37 Shimizu and coworkers developed the perfluoroalkyl-substituted LC triphenylene (32)38 and phthalocyanine (33).39 Aida’s group synthesized an LC metalloporphyrin dyad (34) asymmetrically substituted by polar and non-polar side chains.40
3-2. SIDE CHAINS WITH IONIC MOIETY
Other than the polar side chains described in the preceding section, LC molecules having an ionic unit at the terminal of the alkyl side chain have been reported so far. The LC molecules having ammonium,41 phosphonium,41d sulfonium,42 imidazolium,43 and pyridinium moieties44 have been synthesized. The main driving force of the formation of their LC phases is nanosegregation.45 This phenomenon is similar to the micelles and vesicles in water in the surfactant systems.
Figure 6 shows molecular structure of LC semiconductors bearing ionic moieties at the terminals of the alkyl chains. Funahashi and Kato et al. synthesized a phenylterthiophene derivative (35) having ionic imidazolium units at the terminal of side chains. These compounds formed nanosegregated smectic layer structures, in which ionic units and rigid π-conjugated aromatic cores were separately assembled. In these LC systems, the electrochromism was observed under the application of a DC bias without any electrolytes. In the nanosegregated smectic phases, ions can be transported within the two-dimensional mantles while the electronic charge carriers can also move within the layers made from aromatic cores.46 Fukushima et al. synthesized triphenylene derivatives (36), in which six imidazolium groups and central aromatic core were connected via aliphatic linkers. These compounds exhibited columnar LC phases. Moreover, the triphenylene derivative having the tetrafluoroborate anions as counterparts can be hybridized with ionic liquids and carbon nanotubes.43h,47
4. SELF-ORGANIZATION WITH NON-POLAR SIDE CHAINS
As mentioned in section 2, nanosegregation effect is remarkable in the formation of the LC phases of the molecules bearing polar functional groups. Even in the LC phases of the molecules bearing non-polar side chains, this nanosegregation effect is also significant. Non-polar side chains do not only weaken the strong interaction between π -conjugated cores to induce LC phase, but also promote structural formation by their attractive interaction.
4-1. LINEAR ALKYL CHAINS
Most general flexible units are linear alkyl chains while rigid cores are often heteroaromatic units. Thermal motion of the alkyl chains weaken the strong interaction between the heteroaromatic cores, resulting in the appearance of LC phases and increase in the solubility. On the other hand, heteroaromatic cores are self-organized in soft aggregates by the attractive interaction and nanosegregation of the alkyl chains.
Yasuda and Kato et al. synthesized LC oligothiophenes (37-39) with polycatenar structures, as shown in Figure 7. It should be noted that various LC structures such as miceller cubic (0D), columnar (1D) and smectic (2D) phases were formed due to the nanosegregation between π-conjugated oligothiophene units and alkyl side chains. In addition, they verified the ambipolar carrier transporting properties in the columanr and smectic phases.11c,14g
In the polycatenar LC molecules, nanosegregation effect of alkyl chains should be more remarkable, compared to the classical LC molecules bearing one or two alkyl chains.
Ohta et al. reported an evidence of an attractive interaction between alkyl chains in LC systems. They synthesized octaalkoxy bis(diphenyldioximato) metal complexes (40) and octaalkoxy bis(diphenyldithiolene) metal complexes (41) (Figure 8).48 The alkylated complexes exhibited bathochromic shift in UV-VIS absorption spectra compared to non-alkylated analogues. This result is attributed to the compression of the columnar aggregates by the attractive interaction between the alkyl chains.48c
4-2. BRANCHED ALKYL CHAINS49
Branched alkyl chains are more bulky than the linear alkyl chains. The branched chains have low interfacial free volumes and high surface coverage. Therefore, the aggregation of the molecules bearing the branched alkyl chains should be relatively weakened compared to those bearing linear alkyl chains.
Figure 9 demonstrates the molecular structures of LC semiconductors bearing branched alkyl chains. Funahashi and Kato reported that phenylterthiophene derivative (42) with 3,7,11-trimethyldecyloxy groups exhibits a nematic phase around room temperature.50 This report provides one example of the effectiveness of the branched alkyl groups for the formation of LC phases with the low order. The introduction of the branched aliphatic unit results the appearance of the nematic phase around room temperature. The hole mobility in the nematic phase of the compound was 4×10-4 cm2 V-1 s-1. Recently, Isoda and Tadokoro et al. reported that tetraazanaphthacene (TANC) derivatives (43) bearing the 3,7,11-trimethyldecyloxy or 3,7-dimethyloctyloxy group as branched chains show the smectic phases.51 In spite of the high crystallinity of the TANC core, the compounds surprisingly shows the mesomorphism. The electron mobilities of these LC TANC derivatives are of the order of 10-4 cm2 V-1 s-1.
4-3. CHIRAL ALKYL CHAINS
Among branched alkyl chains, chiral alkyl chains play significant roles in the living body systems. Formation of supramolecular aggregates of chiral molecules for chiral molecular recognition has been main research topics in biology, physics, pharmacy and chemistry. In chiral LC systems, helical structures are formed so that in order to minimize the elastic free energy in the system. The helical structure and helical axis reflect the absolute configuration of the molecules labeled (R) or (S).
When a chiral alkyl chain is introduced to the LC molecule which has a tendency to exhibit a nematic phase, it twists around the axis perpendicular to the director, resulting in the formation of a helical structure spontaneously (Figure 10a). This twisted nematic phase is called as a chiral nematic (N*) or a cholesteric phase. (The name of cholestric phase is derived from the fact that this phase was first discovered for cholesteryl esters.)
In the N* phase, specific selective reflection is observed. The characteristic phenomenon is originated from the helical structure of the N* phase and the helical pitch depends on the temperature of the LC system. Therefore, it has been demonstrated for the application to the thermal sensor. Moreover, the design of the luminescent LC molecules offers materials emitting circularly polarized light (CPL) and organic lasing media.
In the smectic phases, chiral alkyl side chains often generate a helical axis along the layer normal. In the smectic phases in which the director tilts to the layer normal, the director changes periodically along the layer normal (Figure 10b). Such a phase is called as a chiral smectic C (SmC*) phase. This phase exhibits ferroelectricity and the application to high speed displays was attempted.52
Figure 11 shows molecular structures of chiral LC semiconductors. Kelly, O’Neil and coworkers developed a photoluminescent chiral nematic LC material 44, as shown in Figure 11. Compound 44 exhibits glassy N* phase at room temperature and its thin film exhibits a reflection band with a wide wavelength range covering visible light region. The thin film emits high quality CPL fluorescence over a wide wavelength range. They have also studied on the carrier transport properties in the N* phase of the compound.53
Recently, we reported that (R)- and (S)-dimers (45) based on phenylterthiophene skelton exhibit the N* phases. In the mixtures of the (R)- and (S)-enantiomers, the helical pitches were tuned by changing the composition ratio of the both enantiomers. As optimizing the composition so that the reflection band covers the luminescent band of the phenylterthiophene core, the prominent CPL emission was observed. The CPL dissymmetry factor indicating the quality of CPL reaches 1.5. The LC dimers showed an ambipolar carier transport property. Both hole and electron mobilities of the dimers were around 3×10-4 cm2 V-1 s-1.54
Another function of the chirality is breaking the symmetry of the systems. In the ferroelectric phase, a macroscopic polarization is induced by the application of the DC bias and the spontaneous polarization is maintained after switching off the bias. We have recently synthesized the fluorophenylterthiophene derivatives (46) exhibiting the hole transport property in the ferroelectric SmC* phases (Figure 11).55 In this system, we observed an anomalous photovoltaic (APV) effect, which has been reported in very few organic materials. This APV effect is caused by the internal electric field derived from the spontaneous polarization of the ferroelectric materials while the conventional photovoltaic effect is originated from the built-in potential formed at p-n junction.56
As we describes in this section, the introduction of chirality to LC systems leads to the formation of the helical structures in which optical and electronic properties couple to create new opto-electronic functions.
5. SELF-ORGANIZATION WITH SILOXANE MOIETIES
5-1. LINEAR SILOXANE CHAINS
Goodby et al. first reported the LC compounds (47-49) bearing the disiloxane and cage-shaped silsesquioxane units in 1999 (Figure 12).57 In this report, the cyanobiphenyl as the representative mesogen with the side chain modified by the siloxane moieties were synthesized and they studied the influence of the siloxane moieties on their LC properties. The stabilization of LC phases and drops of their melting points were observed. Their mesomorphisms are based on the formation of core-shell structures resulting from the nanosegregation between the siloxane moieties and the rigid biphenyl cores.
In past years, it has been revealed that the linear siloxane chains instead of the alkyl chains are effective to the stabilization of the LC phases. The rotational barrier of the siloxane bond is lower than that of the carbon-carbon bonds of the alkyl groups, and the dimethylsiloxane unit is bulkier than the methylene unit in alkyl chains. Apparently oligosiloxane units should have a tendency to destabilize the formation of LC phases. However, some experimental results described later have indicated that linear siloxane units contribute to the stabilization of the LC phases. This remarkable stabilization of LC phases should be owing to the nanosegregation between dynamic siloxane units and closely aggregated cores. The nanosegregation was driven by difference in the van der Waals interactions between the siloxane units and the π-π interactions between the cores. Based on this idea, we have designed and synthesized some LC π-conjugated materials bearing the siloxane units. As shown in Figure 13, Ferroelectric LC molecules (50-52) bearing a disiloxane or trisiloxane unit have been reported these ferroelectric LC materials exhibit temperature-independent interlayer distances.58 Unlike the conventional ferroelectric LC materials which are insulators, we synthesized ferroelectric LC semiconductor (53) based on a fluorophenylterthiophenes core and observed lowering the transition temperatures by introduction of a disiloxane unit in the system based on the FLC. We observed that the bilayer structure was formed in the SmC* phase of the FLC compound bearing the disiloxane chain due to the nanosegregation between the alkyl units and the siloxane moieties.55
In another LC system, we also observed the stabilization of columnar phases by the introduction of the linear siloxane moieties. Figure 14 shows molecular structures of LC PTCBI derivatives bearing oligosiloxane chains. LC PTCBI derivatives (54, 55) bearing four linear siloxane units were synthesized in our group. The LC PTCBI derivative (55) connected four trisiloxane moieties via swallow tail type linkers has lower melting point than the PTCBIs functionalized with normal alkyl chains. The columnar phases of LC PTCBIs are thermodynamically stable in a wide temperature range including a room temperature. They exhibit high electron mobilities up to 10-1 cm2 V-1 s-1 in the columnar phase at room temperature. In spite of this high electron mobility comparable to those of molecular crystals, the LC phases are soft at room temperature and soluble even in nonpolar solvents. Uniaxially aligned thin films can be produced on friction-transferred substrates by a spin-coating method. This efficient electron mobility should be attributed to a nanosegregated columnar structure. In the LC phase, one-dimensional stacking aggregates of π-conjugated cores is formed and they are surrounded by liquid-like mantle consisting of disordered oligosiloxane chains.59
Moreover, a hybridized LC system with a polar substituent and oligosiloxane units has been reported. The PTCBI derivative (56) bearing two disiloxane units and a triethylene oxide chain was synthesized. This PTCBI derivative exhibits a smectic phase at room temperature, which has a bilayer structure and can form the complex with Li ion maintaining the LC structures.60 In this LC phase of this compound, ion-conductive and electron-conductive layers are assembled separately on nanometer scale through nanosegregation.
5-2. CYCLIC SILOXANE MOIETIES
Not only linear oligosiloxane chains, but cyclic oligosiloxane moieties can also function as side chains of LC molecules. We have demonstrated the introduction of polymerizable siloxane rings to PTCBI and oligothiophene skeletons in order to stabilize the LC structures (Figure 15). The PTCBI derivative (57) bearing the four cyclotetrasiloxane units through the branched linkers exhibit the rectangular columnar disordered (Colrd) phase in a wide temperature range including room temperature. Interestingly, the LC PTCBI derivative transitions around -35 ºC to the LC glass state without crystallization. While maintaining the softness originated from core-shell type supramolecular organization, the electron mobility at room temperature exceeds the order of 10-2 cm2 V-1 s-1.61
Furthermore, the ferroelectric LC fluorophenylterthiophene derivative (58) having a cyclotetrasiloxane unit exhibit smectic phases in a wide temperature range including room temperature. The phase transition temperature was also lowered. This compound maintains LC phase in low-temperature region without crystallization.55 Apparently the cyclotetrasiloxane rings are bulky and should inhibit LC molecular order. However, LC phases are stabilized over wide temperature ranges for oligothiophene and PTCBI derivatives. We can design electronic functional materials which exhibit the LC phases over wide temperature ranges, considering the volume balance between the core, chains and rings.
5-3. CONJUGATED POLYMERS BEARING OLIGOSILOXANE MOIETIES
Recently, some π-conjugated polymers bearing siloxane units have been reported (Figure 16).62 These reports revealed the siloxane units play a crucial role in the improvement of the solubility without inhibiting the aggregation of π-conjugated units. Mori et al. synthesized a solubilized polythiophene (59) bearing the disiloxane moiety at the terminal of the side chain. The polymer has a high head-to-tail regioregularity and high solubility to hexane.62a Bao and coworkers synthesized isoindigo-based polymers bearing a trisiloxane unit (60-62), which exhibited high field-effect mobilities as well as high solubilities in conventional organic solvents.62b Especially, the terthiophene-based polymer (61) shows ambipolar carrier transport property.62c Furthermore, they fabricated bulk-heterojunction organic solar cells using a bithiophene-based polymer (60). The active layer of the cell was composed of the mixture of the polymer (60) and solubilized fullerene derivative (PC71BM), which gave the ideal film morphology leading to the good device performance.62d Oh and Yang’s group synthesized a diketopyrrolopyrrole-selenophene polymer (63) having a trisiloxane unit. They observed the ambipolar carrier transport property in a field effect transistor based on the polymer. The field-effect mobilities of hole and electrons are on the order of 100 cm2 V-1 s-1.62f
These results show that the optimization of side chain unit with siloxane units contributes to the compatibility for the device performance and the processability not only in LC materials but also in conjugated polymers.
6. SUMMARY AND OUTLOOK
LC semiconductors exhibit high carrier mobility as well as solution-processability. Moreover, various electronic and optical functions can be created based on the flexible and dynamic nanostructures formed through nanosegregation. In this sense, design of side chains is more significant than that of π-conjugated cores although main trend of the researches have been concentrated on the design of aromatic cores in this research field. In addition to designs of π-conjugated cores, engineering of side chains of LC molecules should be effective to develop new soft-electronic materials.
References
1. (a) V. I. Minkin, Chem. Rev., 2004, 104, 2751; CrossRef (b) B. S. Lukyanov and M. B. Lukyanova, Chem. Heterocycl. Compd., 2005, 41, 281; CrossRef (c) Z. Zhou and Z. Shen, J. Mater. Chem. C, 2015, 3, 3239; CrossRef (d) T. Suzuki, T. Takeda, E. Ohta, K. Wada, R. Katoono, H. Kawai, and K. Fujiwara, Chem. Rev., 2015, 115, 280; (e) H. Langhals, Heterocycles, 1995, 40, 477; CrossRef (f) J. L. Segura and N. Martín, J. Mater. Chem., 2000, 10, 2403; CrossRef (g) V. Sridharan, P. A. Suryavanshi, and J. C. Menéndez, Chem. Rev., 2011, 111, 7157; CrossRef (h) M. A. Weaver and L. Shuttleworth, Dyes Pigments, 1982, 3, 81; CrossRef (i) P. Przybylski, A. Huczynski, K. Pyta, B. Brzezinski, and F. Bartl, Curr. Org. Chem., 2009, 13, 124; CrossRef (j) M. Wainwright and R. M. Giddens, Dyes Pigments, 2003, 57, 245. CrossRef
2. (a) E. S. Gil and S. M. Hudson, Prog. Polym. Sci., 2004, 29, 1173; CrossRef (b) H. Otsuka, Y. Nagasaki, and K. Kataoka, Adv. Drug Deliv. Rev., 2003, 55, 403; CrossRef (c) R. A. Segalman, Mater. Sci. Eng. R Rep., 2005, 48, 191; CrossRef (d) M. R. Bockstaller, R. A. Mickiewicz, and E. L. Thomas, Adv. Mater., 2005, 17, 1331; CrossRef (e) H.-A. Klok and S. Lecommandoux, Adv. Mater., 2001, 13, 1217. CrossRef
3. (a) O. Gronwald, E. Snip, and S. Shinkai, Curr. Opin. Colloid Interface Sci., 2002, 7, 148; CrossRef (b) Y. Noda, Y. Hayashi, and K. Ito, J. Appl. Polym. Sci., 2014, 131, 40509. CrossRef
4. (a) T. Kato, N. Mizoshita, and K. Kishimoto, Angew. Chem. Int. Ed., 2006, 45, 38; CrossRef (b) M. Funahashi, H. Shimura, M. Yoshio, and T. Kato, Struct. Bond., 2008, 128, 151; CrossRef (c) T. Kato, T. Yasuda, Y. Kamikawa, and M. Yoshio, Chem. Commun., 2009, 729; CrossRef (d) M. Funahashi, J. Mater. Chem. C, 2014, 2, 7451. CrossRef
5. (a) D. Adam, F. Closs, T. Frey, D. Funhoff, D. Haarer, H. Ringsdorf, P. Schuhmacher, and K. Siemensmeyer, Phys. Rev. Lett., 1993, 70, 457; CrossRef (b) N. Boden, R. J. Bushby, J. Clements, B. Movaghar, K. J. Donovan, and T. Kreouzis, Phys. Rev. B., 1995, 52, 13274; CrossRef (c) A. M. van de Craats, J. M. Warman, A. Fechtenkötter, J. B. Brand, M. A. Harbison, and K. Müllen, Adv. Mater., 1999, 11, 1469; CrossRef (d) M. Ichihara, A. Suzuki, K. Hatsusaka, and K. Ohta, Liq. Cryst., 2007, 34, 555; CrossRef (e) K. Ban, K. Nishikawa, K. Ohta, A. M. van de Craats, J. M. Warman, I. Yamamoto, and H. Shirai, J. Mater. Chem., 2001, 11, 321; CrossRef (f) A. Demenev, S. H. Eichhorn, T. Taerum, D. Perepichka, S. Patwardhan, F. C. Grozema, L. D. A. Siebbeles, and R. Klenkler, Chem. Mater., 2010, 22, 1420; CrossRef (g) I. Bleyl, C. Erdelen, H.-W. Schmidt, and D. Haarer, Philos. Mag. B, 1999, 79, 463; CrossRef (h) J. Simmerer, B. Glüsen, W. Paulus, A. Kettner, P. Schuhmacher, D. Adam, K.-H. Etzbach, K.Siemensmeyer, J. H. Wendorf, H. Ringsdorf, and D. Haarer, Adv. Mater., 1996, 8, 815; CrossRef (i) D. Adam, P. Schumacher, J. Simmerer, L. Haussling, K. Siemensmeyer, K. H. Etzbach, H. Ringsdorf, and D. Haarer, Nature, 1994, 371, 141. CrossRef
6. (a) M. Funahashi and J. Hanna, Phys. Rev. Lett., 1997, 78, 2184; CrossRef (b) M. Funahashi and J. Hanna, Appl. Phys. Lett., 1997, 71, 602; CrossRef (c) M. Funahashi and J. Hanna, Appl. Phys. Lett., 1998, 73, 3733; CrossRef (d) M. Funahashi and J. Hanna, Jpn. J. Appl. Phys., 1999, 38, L132; CrossRef (e) K. Kogo, H. Maeda, H. Kato, M. Funahashi, and J. Hanna, Appl. Phys. Lett., 1999, 75, 3348; CrossRef (f) H. Zhang and J. Hanna, J. Phys. Chem. B, 1999, 103, 7429. CrossRef
7. F. Würthner, C. R. Saha-Möller, B. Fimmel, S. Ogi, P. Leowanawat, and D. Schmidt, Chem. Rev., 2015, in press.
8. (a) C. W. Struijk, A. B. Sieval, J. E. J. Dakhorst, M. van Dijk, P. Kimkes, R. B. M. Koehorst, H. Donker, T. J. Schaafsma, S. J. Picken, A. M. van de Craats, J. M. Warman, H. Zuilhof, and E. J. R. Sudhölter, J. Am. Chem. Soc., 2000, 122, 11057; CrossRef (b) J. Y. Kim and A. J. Bard, Chem. Phys. Lett., 2004, 383, 11; CrossRef (c) J. Y. Kim, I. J. Chung, Y. C. Kim, and J.-W. Yu, Chem. Phys. Lett., 2004, 398, 367; CrossRef (d) Z. An, J. Yu, S. C. Jones, S. Barlow, S. Yoo, B. Domercq, P. Prins, L. D. A. Siebbeles, B. Kippelen, and S. R. Marder, Adv. Mater., 2005, 17, 2580; CrossRef (e) Z. Chen, V. Stepanenko, V. Dehm, P. Prins, L. D. A. Siebbeles, J. Seibt, P. Marquetand, V. Engel, and F. Würthner, Chem. Eur. J., 2007, 13, 436; CrossRef (f) V. Duzhko, E. Aqad, M. R. Imam, M. Peterca, V. Percec, and K. D. Singer, Appl. Phys. Lett., 2008, 92, 113312; CrossRef (g) M.-A. Muth, G. Gupta, A. Wicklein, M. Carrasco-Orozco, T. Thurn-Albrecht, and M. Thelakkat, J. Phys. Chem. C, 2014, 118, 92; CrossRef (h) J. A. Quintana, J. M. Villalvilla, A. de la Peňa, J. L. Segula, and M. A. Díaz-García, J. Phys. Chem. C, 2014, 118, 26577. CrossRef
9. (a) F. Würthner, C. Thalacker, S. Diele, and C. Tschierske, Chem. Eur. J., 2001, 7, 2245; CrossRef (b) J. van Herrikhuyzen, A. Syamakumari, A. P. H. J. Schenning, and E. W. Meijer, J. Am. Chem. Soc., 2004, 126, 10021; CrossRef (c) Z. Chen, U. Baumeister, C. Tschierske, and F. Würthner, Chem. Eur. J., 2007, 13, 450; CrossRef (d) A. Wicklein, A. Lang, M. Muth, and M. Thelakkat, J. Am. Chem. Soc., 2009, 131, 14442. CrossRef
10. (a) F. S. Kim, G. Ren, and S. A. Jenekhe, Chem. Mater., 2011, 23, 682; CrossRef (b) M. O'Neill and S. M. Kelly, Adv. Mater., 2011, 23, 566; CrossRef (c) A. M. van de Craats, J. M. Warman, H. Hasebe, R. Naito, and K. Ohta, J. Phys. Chem. B, 1997, 101, 9224; CrossRef (d) H. Fujikake, T. Murashige, M. Sugibayashi, and K. Ohta, Appl. Phys. Lett., 2004, 85, 3474; CrossRef (e) J. C. Swarts, E. H. G. Langner, N. Krokeide-Hove, and M. J. Cook, J. Mater. Chem., 2001, 11, 434; CrossRef (f) Y. Miyake, Y. Shiraiwa, K. Okada, H. Monobe, T. Hori, N. Yamasaki, H. Yoshida, M. J. Cook, A. Fujii, and M. Ozaki, Appl. Phys. Express, 2011, 4, 021604; CrossRef (g) T. Hori, N. Fukuoka, T. Masuda, Y. Miyake, H. Yoshida, A. Fujii, Y. Shimizu, and M. Ozaki, Sol. Energy Mater. Sol. Cells, 2011, 95, 3087; (h) S. Tanaka, T. Sakurai, Y. Honsho, A. Saeki, S. Seki, K. Kato, M. Takata, A. Osuka, and T. Aida, Chem. Eur. J., 2012, 18, 10554; CrossRef (i) S. Sengupta, S. Uemura, S. Patwardhan, V. Huber, F. C. Grozema, L. D. A. Siebbeles, U. Baumeister, and F. Würthner, Chem. Eur. J., 2011, 17, 5300; CrossRef (j) K. Oikawa, H. Monobe, K. Nakayama, T. Kimoto, K. Tsuchiya, B. Heinrich, D. Guillon, Y. Shimizu, and M. Yokoyama, Adv. Mater., 2007, 19, 1864; CrossRef (k) K. Tokunaga, H. Iino, and J. Hanna, J. Phys. Chem. B, 2007, 111, 12041; CrossRef (l) K. Tokunaga, H. Iino, and J. Hanna, Mol. Cryst. Liq. Cryst., 2009, 510, 241.
11. (a) H.-C. Chang, K. Komasaka, K. Kishida, T. Shiozaki, T. Ohmori, T. Matsumoto, A. Kobayashi, M. Kato, and S. Kitagawa, Inorg. Chem., 2011, 50, 4279; CrossRef (b) H.-C. Chang, T. Shiozaki, A. Kamata, K. Kishida, T. Ohmori, D. Kiriya, T. Yamauchi, H. Furukawa, and S. Kitagawa, J. Mater. Chem., 2007, 17, 4136; CrossRef (c) T. Yasuda, H. Ooi, J. Morita, Y. Akama, K. Minoura, M. Funahashi, T. Shimomura, and T. Kato, Adv. Funct. Mater., 2009, 19, 411. CrossRef
12. M. Mitani, S. Yamane, M. Yoshio, M. Funahashi, and T. Kato, Mol. Cryst. Liq. Cryst., 2014, 594, 1. CrossRef
13. R. Azumi, Ekisho, 2006, 10, 52.
14. (a) I. McCulloch, W. Zhang, M. Heeney, C. Bailey, M. Giles, D. Graham, M. Shkunov, D. Sparrowe, and S. Tierney, J. Mater. Chem., 2003, 13, 2436; CrossRef (b) D. Byron, A. Matharu, R. Wilson, and G. Wright, Mol. Cryst. Liq. Cryst., 1995, 265, 61; CrossRef (c) F. Garnier, R. Hajlaoui, A. El Kassmi, G. Horowitz, L. Laigre, W. Porzio, M. Armanini, and F. Provasoli, Chem. Mater., 1998, 10, 3334; CrossRef (d) R. Azumi, G. Götz, and P. Bäuerle, Synth. Met., 1999, 101, 544; CrossRef (e) S. Ponomarenko and S. Kirchmeyer, J. Mater. Chem., 2003, 13, 197; CrossRef (f) H. Wada, T. Taguchi, M. Goto, T. Kambayashi, T. Mori, K. Ishikawa, and H. Takezoe, Chem. Lett., 2006, 35, 280; CrossRef (g) T. Yasuda, K. Kishimoto, and T. Kato, Chem. Commun., 2006, 3399. CrossRef
15. (a) J. Leroy, J. Levin, S. Sergeyev, and Y. Geerts, Chem. Lett., 2006, 35 166; CrossRef (b) J. Leroy, N. Boucher, S. Sergeyev, M. Sferrazza, and Y. Geerts, Eur. J. Org. Chem., 2007, 1256; CrossRef (c) N. Boucher, J. Leroy, S. Sergeyev, E. Pouzet, V. Lemaur, R. Lazzaroni, J. Cornil, Y. H. Geerts, and M. Sferrazza, Synth. Met., 2009, 159, 1319; CrossRef (d) A. J. J. M. van Breemen, P. T. Herwig, C. H. T. Chlon, J. Sweelssen, H. F. M. Schoo, S. Setayesh, W. M. Hardeman, C. A. Martin, D. M. de Leeuw, J. J. P. Valeton, C. W. M. Bastiaansen, D. J. Broer, A. R. Popa-Merticaru, and S. C. J. Meskers, J. Am. Chem. Soc., 2006, 128, 2336. CrossRef
16. (a) M. Funahashi, F. Zhang, and N. Tamaoki, Adv. Mater., 2007, 19, 353; CrossRef (b) F. Zhang, M. Funahashi, and N. Tamaoki, Appl. Phys. Lett., 2007, 91, 063515; CrossRef (c) M. Funahashi, T. Ishii, and A. Sonoda, ChemPhysChem, 2013, 14, 2750. CrossRef
17. T. Izawa, E. Miyazaki, and K. Takimiya, Adv. Mater., 2008, 20, 3388. CrossRef
18. (a) A. Kumatani, C. Liu, Y. Li, P. Darmawan, K. Takimiya, T. Minari, and K. Tsukagoshi, Sci. Rep., 2012, 2, 393; CrossRef (b) Y. Li, C. Liu, A. Kumatani, P. Darmawan, T. Minari, and K. Tsukagoshi, Org. Electron., 2012, 13, 264; CrossRef (c) Y. Li, C. Liu, Y. Xu, T. Minari, P. Darmawan, and K. Tsukagoshi, Org. Electron., 2012, 13, 815; CrossRef (d) Y. Yuan, G. Giri, A. L. Ayzner, A. P. Zoombelt, S. C. B. Mannsfeld, J. Chen, D. Nordlund, M. F. Toney, J. Huang, and Z. Bao, Nat. Commun., 2014, 5, 3005.
19. M. J. Kang, T. Yamamoto, S. Shinamura, E. Miyazaki, and K. Takimiya, Chem. Sci., 2010, 1, 179. CrossRef
20. (a) Y. S. Yang, T. Yasuda, H. Kakizoe, H. Mieno, H. Kino, Y. Tateyama, and C. Adachi, Chem. Commun., 2013, 49, 6483; CrossRef (b) H. Mieno, T. Yasuda, Y. S. Yang, and C. Adachi, Chem. Lett., 2014, 43, 293. CrossRef
21. B. Košata, V. Kozmik, J. Svoboda, V. Novotná, P. Vanĕk, and M. Glogarová, Liq. Cryst., 2003, 30, 603. CrossRef
22. H. Iino and J. Hanna, Adv. Mater., 2011, 23, 1748. CrossRef
23. J. M. Adhikari, K. Vakhshouri, B. D. Calitree, A. Hexemer, M. A. Hickner, and E. D. Gomez, J. Mater. Chem. C, 2015, 3, 8799. CrossRef
24. (a) H. Iino, T. Kobori, and J. Hanna, J. Non-Cryst. Solids, 2012, 358, 2516; CrossRef (b) H. Iino, T. Usui, and J. Hanna, Nat. Commun., 2015, 6, 6828; CrossRef (c) S. Inoue, H. Minemawari, J. Tsutsumi, M. Chikamatsu, T. Yamada, S. Horiuchi, M. Tanaka, R. Kumai, M. Yoneya, and T. Hasegawa, Chem. Mater., 2015, 27, 3809; CrossRef (d) S. Méry, D. Haristoy, J.-F. Nicoud, D. Guillon, S. Diele, H. Monobe, and Y. Shimizu, J. Mater. Chem., 2002, 12, 37. CrossRef
25. (a) A. C. Arias, J. D. MacKenzie, and I. McCulloch, Chem. Rev., 2010, 110, 3; CrossRef (b) C. R. Newman, C. D. Frisbie, D. A. da Silva Filho, J.-L. Brédas, P. C. Ewbank, and K. R. Mann, Chem. Mater., 2004, 16, 4436. CrossRef
26. (a) B. Roy, N. De, and K. C. Majumdar, Chem. Eur. J., 2012, 189, 14560; CrossRef (b) E. Yashima, K. Maeda, H. Iida, Y. Furusho, and K. Nagai, Chem. Rev., 2009, 109, 6102; CrossRef (c) D. Janietz, J. Mater. Chem. C, 1998, 8, 265; (d) T. Kato, N. Mizoshita, and K. Kanie, Macromol. Rapid Commun., 2001, 22, 797; CrossRef (e) D. J. Broer, C. M. W. Bastiaansen, M. G. De bije, and A. P. H. J. Schenning, Angew. Chem. Int. Ed., 2012, 51, 7102; CrossRef (f) H. Li, J. Choi, and T. Nakanishi, Langmuir, 2013, 29, 5394. CrossRef
27. (a) S. Singh, Phys. Rep., 2000, 324, 107; CrossRef (b) C. Zannoni, J. Mater. Chem., 2001, 11, 2637. CrossRef
28. (a) D. Vorländer, Ber. Dtsch. Chem. Ges., 1929, 62, 2831; CrossRef (b) G. Pelzl, I. Wirth, and W. Weissflog, Liq. Cryst., 2001, 28, 969; CrossRef (c) H. Takezoe and Y. Takanishi, Jpn. J. Appl. Phys., 2006, 45, 597; CrossRef (d) M. Sawamura, K. Kawai, Y. Matsuo, K. Kanie, T. Kato, and E. Nakamura, Nature, 2002, 419, 702. CrossRef
29. (a) J. Charvolin, J. Chim. Phys., 1983, 80, 15; (b) C. Tschierske, Angew. Chem. Int. Ed., 2013, 52, 8828; CrossRef (c) J. P. F. Lagerwall and F. Giesselmann, ChemPhysChem, 2006, 7, 20; CrossRef (d) C. Tschierske, Isr. J. Chem., 2012, 52, 935; CrossRef (e) J. W. Goodby, E. J. Davis, R. J. Mandle, and S. J. Cowling, Isr. J. Chem., 2012, 52, 863. CrossRef
30. (a) R. Duran, P. Gremain, D. Guillon, and A. Skoulios, Mol. Cryst. Liq. Cryst. Lett., 1986, 3, 23; (b) N. H. Tinh, J. Malthete, and C. Destrade, Mol. Cryst. Liq. Cryst. Lett., 1985, 2, 133; (c) J.-K. Kim, M.-K. Hong, J.-H. Ahn, and M. Lee, Angew. Chem. Int. Ed., 2005, 44, 328; CrossRef (d) F. Hildebrandt, J. A. Schröter, C. Tschierske, R. Festag, M. Wittenberg, and J. H. Wendorff, Adv. Mater., 1997, 9, 564; CrossRef (e) N.-K. Oh, W.-C. Zin, J.-H. Im, J.-H. Ryu, and M. Lee, Chem. Commun., 2004, 1092. CrossRef
31. (a) M. Lee, B.-K. Cho, Y.-G. Jang, and W.-C. Zin, J. Am. Chem. Soc., 2000, 122, 7449; CrossRef (b) M. Lee, B.-K. Cho, K. J. Ihn, W.-K. Lee, N.-K. Oh, and W.-C. Zin, J. Am. Chem. Soc., 2001, 123, 4647. CrossRef
32. (a) K. Hoshino, K. Kanie, T. Ohtake, T. Mukai, M. Yoshizawa, S. Ujiie, H. Ohno, and T. Kato, Macromol. Chem. Phys., 2002, 203, 1547; CrossRef (b) B. Bilgin-Eran, C. Tschierske, S. Diele, and U. Baumeister, J. Mater. Chem., 2006, 16, 1145; CrossRef (c) B. Bilgin-Eran, Ç. Yörür, C. Tschierske, M. Prehm, and U. Baumeister, J. Mater. Chem., 2007, 17, 2319; CrossRef (d) N. Terasawa, H. Monobe, K. Kiyohara, and Y. Shimizu, Chem. Lett., 2003, 32, 214; CrossRef (e) Y. Yang, H. Li, and J. Wen, Liq. Cryst., 2007, 34, 1167; CrossRef (f) N. Terasawa, H. Monobe, and K. Kiyohara, Liq. Cryst., 2007, 34, 311. CrossRef
33. (a) S. Pensec and F.-G. Tournilhac, Chem. Commun., 1997, 441; CrossRef (b) M. Murase, Y. Takanishi, I. Nishiyama, A. Yoshizawa, and J. Yamamoto, RSC Adv., 2015, 5, 215.
34. M. Yoneya, Chem. Rec., 2011, 11, 66. CrossRef
35. (a) M. Prehm, G. Götz, P. Bäuerle, F. Liu, X. Zeng, G. Ungar, and C. Tschierske, Angew. Chem. Int. Ed., 2007, 46, 7856; CrossRef (b) W. Bu, H. Gao, X. Tan, X. Dong, X. Cheng, M. Prehm, and C. Tschierske, Chem. Commun., 2013, 49, 1756. CrossRef
36. A. P. H. J. Schenning, A. F. M. Kilbinger, F. Biscarini, M. Cavallini, H. J. Cooper, P. J. Derrick, W. J. Feast, R. Lazzaroni, P. Leclère, L. A. McDonell, E. W. Meijer, and S. C. J. Meskers, J. Am. Chem. Soc., 2002, 124, 1269. CrossRef
37. (a) R. A. Cormier and B. A. Gregg, Chem. Mater., 1998, 10, 1309; CrossRef (b) B. A. Gregg and R. A. Cormier, J. Am. Chem. Soc., 2001, 123, 7959; CrossRef (c) S.-G. Liu, G. Sui, R. A. Cormier, R. M. Leblanc, and B. A. Gregg, J. Phys. Chem. B, 2002, 106, 1307; CrossRef (d) S.-G. Chen, P. Stradins, and B. A. Gregg, J. Phys. Chem. B, 2005, 109, 13451; CrossRef (e) B. A. Gregg and M. E. Kose, Chem. Mater., 2008, 20, 5235. CrossRef
38. Y. Miyake, A. Fujii, M. Ozaki, and Y. Shimizu, Synth. Met., 2009, 159, 875. CrossRef
39. L. Sosa-Vargas, F. Nekelson, D. Okuda, M. Takahashi, Y. Matsuda, Q.-D. Dao, H. Yoshida, A. Fujii, M. Ozaki, and Y. Shimizu, J. Mater. Chem. C, 2015, 3, 1757. CrossRef
40. T. Sakurai, K. Shi, H. Sato, K. Tashiro, A. Osuka, A. Saeki, S. Seki, S. Tagawa, S. Sasaki, H. Masunaga, K. Osaka, M. Takata, and T. Aida, J. Am. Chem. Soc., 2008, 130, 13812. CrossRef
41. (a) F. Tittarelli, P. Masson, and A. Skoulios, Liq. Cryst., 1997, 22, 721; CrossRef (b) M. Huskic and M. Zigon, Liq. Cryst., 2002, 29, 1217; CrossRef (c) S. Ujiie and Y. Yano, Chem. Commun., 2000, 79; CrossRef (d) T. Ichikawa, M. Yoshio, A. Hamasaki, S. Taguchi, F. Liu, X.–B. Zeng, G. Ungar, H. Ohno, and T. Kato, J. Am. Chem. Soc., 2012, 134, 2634. CrossRef
42. (a) T. Matsumoto, T. Ichikawa, J. Sakuda, T. Kato, and H. Ohno, Bull. Chem. Soc. Jpn., 2014, 87, 792; CrossRef (b) T. Ichikawa, T. Kato, and H. Ohno, J. Am. Chem. Soc., 2012, 134, 11354. CrossRef
43. (a) M. Yoshio, T. Mukai, H. Ohno, and T. Kato, J. Am. Chem. Soc., 2004, 126, 994; CrossRef (b) M. Yoshio, T. Kato, T. Mukai, M. Yoshizawa, and H. Ohno, Mol. Cryst. Liq. Cryst., 2004, 413, 2235; CrossRef (c) M. Yoshio, T. Kagata, K. Hoshino, T. Mukai, H. Ohno, and T. Kato, J. Am. Chem. Soc., 2006, 128, 5570; CrossRef (d) M. Yoshio, T. Ichikawa, H. Shimura, T. Kagata, A. Hamasaki, T. Mukai, H. Ohno, and T. Kato, Bull. Chem. Soc. Jpn., 2007, 80, 1836; CrossRef (e) D. Högberg. B. Soberats, S. Uchida, M. Yoshio, L. Kloo, H. Segawa, and T. Kato, Chem. Mater., 2014, 26, 6496; CrossRef (f) J. Sakuda, E. Hosono, M. Yoshio, T. Ichikawa, T. Matsumoto, H. Ohno, H. Zhou, and T. Kato, Adv. Funct. Mater., 2015, 25, 1206; CrossRef (g) H. Yoshizawa, T. Mihara, and N. Koide, Mol. Cryst. Liq. Cryst., 2004, 423, 61; CrossRef (h) J. Motoyanagi, T. Fukushima, and T. Aida, Chem. Commun., 2005, 101. CrossRef
44. (a) D. Navarro-Rodriguez, Y. Frere, P. Gramain, D. Guillon, and A. Skoulios, Liq. Cryst., 1991, 9, 321; CrossRef (b) E. Bravo-Grimaldi, D. Navarro-Rodriguez, A. Skoulios, and D. Guillon, Liq. Cryst., 1996, 20, 393; CrossRef (c) L. Cui, V. Sapagovas, and G. Lattermann, Liq. Cryst., 2002, 29, 1121; CrossRef (d) V. Hessel, H. Ringsdorf, R. Festag, and J. H. Wendorff, Macromol. Chem. Rapid Commun., 1993, 14, 707; CrossRef (e) S. Kumar and S. K. Pal, Tetrahedron Lett., 2005, 46, 4127. CrossRef
45. K. Binnemans, Chem. Rev., 2005, 105, 4148. CrossRef
46. (a) S. Yazaki, M. Funahashi, and T. Kato, J. Am. Chem. Soc., 2008, 130, 13206; CrossRef (b) S. Yazaki, M. Funahashi, J. Kagimoto, H. Ohno, and T. Kato, J. Am. Chem. Soc., 2010, 132, 7702. CrossRef
47. J. J. Lee, A. Yamaguchi, M. A. Alam, Y. Yamamoto, T. Fukushima, K. Kato, M. Takata, N. Fujita, and T. Aida, Angew. Chem. Int. Ed., 2012, 51, 8490. CrossRef
48. (a) K. Ohta, R. Higashi, M. Ikejima, I. Yamamoto, and N. Kobayashi, J. Mater. Chem., 1998, 8, 1979; CrossRef (b) K. Ohta, H. Hasebe, M. Moriya, T. Fujimoto, and I. Yamamoto, Mol. Cryst. Liq. Cryst., 1991, 208, 33; CrossRef (c) K. Ohta, Y. Inagaki-Oka, H. Hasebe, and I. Yamamoto, Polyhedron, 2000, 19, 267. CrossRef
49. M. J. Hollamby and T. Nakanishi, J. Mater. Chem.C, 2013, 1, 6178. CrossRef
50. M. Nuita, J. Sakuda, Y. Hirai, M. Funahashi, and T. Kato, Chem. Lett., 2011, 40, 412. CrossRef
51. K. Isoda, T. Abe, M. Funahashi, and M. Tadokoro, Chem. Eur. J., 2014, 20, 7232. CrossRef
52. I. Nishiyama, Chem. Rec., 2009, 9, 340. CrossRef
53. (a) S. R. Farrar, A. E. A. Contoret, M. O’Neill, J. E. Nicholls, G. J. Richards, and S. M. Kelly, Phys. Rev. B, 2002, 66, 125107; CrossRef (b) K. L. Woon, M. O’Neill, G. J. Richards, M. P. Aldred, S. M. Kelly, and A. M. Fox, Adv. Mater., 2003, 15, 1555; CrossRef (c) K. L. Woon, M. O’Neill, P. Vlachos, M. P. Aldred, and S. M. Kelly, Liq. Cryst., 2005, 32, 1191; CrossRef (d) K. L. Woon, M. O’Neill, G. J. Richards, M. P. Aldred, and S. M. Kelly, J. Opt. Soc. Am. A, 2005, 22, 760; CrossRef (e) K. L. Woon, M. O’Neill, G. J. Richards, M. P. Aldred, and S. M. Kelly, Phys. Rev. E, 2005, 71, 041706. CrossRef
54. T. Hamamoto and M. Funahashi, J. Mater. Chem. C, 2015, 3, 6891. CrossRef
55. Y. Funatsu, A. Sonoda, and M. Funahashi, J. Mater. Chem. C, 2015, 3, 1982. CrossRef
56. K. T. Butler, J. M. Frost, and A. Walsh, Energ. Environ. Sci., 2015, 8, 838. CrossRef
57. G. H. Mehl, A. J. Thornton, and J. W. Goodby, Mol. Cryst Liq. Cryst., 1999, 332, 455. CrossRef
58. (a) J. P. F. Lagarwall and F. Giesselmann, ChemPhysChem, 2006, 7, 20; CrossRef (b) J. Naciri, J. Ruth, G. Crawford, R. Shashidhar, and B. R. Ratna, Chem. Mater., 1995, 7, 1397; CrossRef (c) M. Spector, P. Heiney, J. Naciri, B. Weslowski, D. Holt, and R. Shashidhar, Phys. Rev. E, 2000, 61, 1579; CrossRef (d) J. V. Selinger, P. J. Collings, and R. Shashidhar, Phys. Rev. E, 2001, 64, 061705; CrossRef (e) O. Panarina, Y. Panarin, J. Vij, M. Spector, and R. Shashidhar, Phys. Rev. E, 2003, 67, 051709; CrossRef (f) P. J. Collings, B. R. Ratna, and R. Shashidhar, Phys. Rev. E, 2003, 67, 021705; CrossRef (g) D. Nonnenmacher, M. A. Osipov, J. C. Roberts, R. P. Lemieux, and F. Giesselmann, Phys. Rev. E, 2010, 82, 031703; CrossRef (h) D. Nonnenmacher, R. P. Lemieux, M. A. Osipov, and F. Giesselmann, ChemPhysChem, 2014, 15, 1368. CrossRef
59. (a) M. Funahashi and A. Sonoda, Org. Electron., 2012, 13, 1633; CrossRef (b) M. Funahashi and A. Sonoda, J. Mater. Chem., 2012, 22, 25190. CrossRef
60. M. Funahashi and A. Sonoda, Dalton Trans., 2013, 42, 15987. CrossRef
61. M. Funahashi, M. Yamaoka, K. Takenami, and A. Sonoda, J. Mater. Chem. C, 2013, 1, 7872. CrossRef
62. (a) A. Mori, K. Ide, S. Tamba, S. Tsuji, Y. Toyomori, and T. Yasuda, Chem. Lett., 2014, 43, 640; CrossRef (b) J. Mei, D. H. Kim, A. L. Ayzner, M. F. Toney, and Z. Bao, J. Am. Chem. Soc., 2011, 133, 20130; CrossRef (c) H. Li, J. Mei, A. L. Ayzner, M. F. Toney, J. B.-H. Tok, and Z. Bao, Org. Electron., 2012, 13, 2450; CrossRef (d) D. H. Kim, A. L. Ayzner, A. L. Appleton, K. Schmidt, J. Mei, M. F. Toney, and Z. Bao, Chem. Mater., 2013, 25, 431; CrossRef (e) J. Mei, H.-C. Wu, Y. Diao, A. Appleton, H. Wang, Y. Zhou, W.-Y. Lee, T. Kurosawa, W.-C. Chen, and Z. Bao, Adv. Funct. Mater., 2015, 25, 3455; CrossRef (f) J. Lee, A-R. Han, J. Kim, Y. Kim, J. H. Oh, and C. Yang, J. Am. Chem. Soc., 2012, 134, 20713. CrossRef