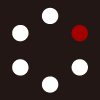
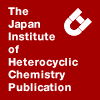
HETEROCYCLES
An International Journal for Reviews and Communications in Heterocyclic ChemistryWeb Edition ISSN: 1881-0942
Published online by The Japan Institute of Heterocyclic Chemistry
e-Journal
Full Text HTML
Received, 13th November, 2015, Accepted, 15th January, 2016, Published online, 28th January, 2016.
DOI: 10.3987/REV-15-830
■ Total Biosynthesis of Fungal Indole Diterpenes Using Cell Factories
Atsushi Minami, Chengwei Liu, and Hideaki Oikawa*
Division of Chemistry, Graduate School of Sceince, Hokkaido University, Kita 10 Nishi 8, Kita-ku, Sapporo, Hokkaido 060-0810, Japan
Abstract
Reconstitution of biosynthetic genes in a heterologous host is a newly emerging method for synthesis of fungal secondary metabolites. Application of this method to indole diterpenes is described. We have successfully elucidated all of the individual enzymatic steps and produced four representative indole diterpenes, paspaline, paxilline, aflatrem, and penitrem, at a yield of ~100 mg/L. These results confirmed that the Aspergillus oryzae expression system is highly reliable for elucidation of biosynthetic pathways, even those involving 17 enzymatic reactions.CONTENTS
1. Introduction
2. Expression system of biosynthetic genes in Aspergillus oryzae
3. Indole diterpenes produced by various fungi
4. Enzymatic synthesis of fungal indole diterpenes
4-1. Paspaline
4-2. Paxilline
4-3. Aflatrem
4-4. Penitrem
5. Conclusion
1. Introduction
In 1917, Robinson reported outstanding total synthesis of tropinone.1 He carefully chose three simple starting materials to set up the sequential reactions (condensation to form iminium ion, Mannich reactions, and decarboxylations), as shown in Scheme 1. Intriguingly, he envisioned the occurrence of the reactions in a cell under physiological conditions, and used starting materials that are likely present in plant cells. This method of synthesis incorporates a biogenetic viewpoint that greatly simplifies the route for synthesis of tropinone. Living organisms synthesize numerous natural products, such as tropinone, from simple metabolites using biosynthetic enzymes. Recent progress in the biosynthesis of natural products provides a basic understanding of the biosynthetic pathways of representative natural products such as polyketides, terpenoids, and alkaloids including nonribosomal and ribosomal peptides at the enzymatic level. In microorganisms, biosynthetic genes are usually found in clusters on the chromosome. Recent rapid progress in DNA sequencing technology has produced large publically available databases of genomic data. Web-based search tools have enabled identification of the gene clusters for biosynthesis of many natural products. Thus, using this information, we can basically synthesize any type of natural products if we can find a method to express gene clusters. In addition, the Web tools also reveal a vast number of natural product gene clusters, most of which have yet to be characterized. Contrary to previous predictions, this finding suggests that discovery of novel natural products may be possible by expression of these dormant gene clusters.
Natural products have been isolated using bioassay-guided screening in many cases. Conventional chemical synthesis is an alternative method for supplying natural products. In addition, as described, reconstitution of biosynthetic gene clusters in heterologous hosts has become an attractive method to produce natural products. Since 2010, we have investigated the enzymatic synthesis of fungal natural products by using gene cluster data in the Aspergillus oryzae expression system. We and others have successfully applied this system to produce various types of natural products.2-8 In this review, we introduce recent efforts for the enzymatic total biosynthesis of indole diterpenes.
2. Expression system of biosynthetic genes in ASPERGILLUS oryzae
From more than a thousand years, the fungus A. oryzae has been used in Japan to produce fermented products, such as sake and soy sauce, at the industrial scale. Following development of genetic engineering methods, strains of A. oryzae that can secrete large amounts of proteins have been used for industrial enzyme production.9,10 Among the various fungal expression systems available, such as the standard host A. nidulans, one of the most effective system is expression under the starch-inducible α-amylase promoter in strains of A. oryzae lacking the ability to produce most secondary metabolites.
The high level of protein expression in this fungus is dependent on a powerful inducible promoter, such as PamyB, which is regulated by malto-oligosaccharides (Figure 1).11 The plasmid pTAex3 consists of the amyB expression cassette (the PamyB promoter and the TamyB terminator) and an argB selectable marker.12 Because this promoter is so robust, heterologous gene expression in A. oryzae has been used for the functional analysis of individual biosynthetic genes such as the multifunctional gene polyketide synthase which is involved in the production of fungal secondary metabolites. During the transformation process, biosynthetic genes are randomly integrated into the chromosome, thus requiring screening for transformants that show a good production (~100 mg/kg, yields are often higher in solid medium than in liquid medium).
Transformed cells harboring a target gene are usually selected using markers of antibiotic resistance, such as hyglomycin or zeocin. However, A. oryzae is naturally resistant to various antibiotics, probably due to the presence of a high number of transporter genes. Therefore, several auxotrophic mutants of A. oryzae are used as hosts together with vectors having selectable markers complemented by argB (for an arginine auxotroph), pyrG gene (for a uridine auxotroph), sC gene (for a methionine auxotroph), adeA gene (for an adenine auxotroph) and niaD gene (for a nitrate assimilation mutant) (Figure 1). A few antibiotic resistant markers, including pPTRI (pyrithiamine), are also used for introducing target genes.
Since 2010, in our group, the use of the A. oryzae expression system has been extended for reconstitution of the biosynthetic machinery for fungal secondary metabolites (Figure 2). Multiple vectors are required to introduce all of the biosynthetic genes in the clusters. To examine the expression of multiple natural product biosynthetic genes, we selected an expression system using the quadruple-auxotrophic host Aspergillus oryzae NSAR1 (niaD–, sC–, ΔargB, adeA–) developed by Kitamoto et al.13 This host strain can accept multiple vectors such as pTAex3,12 pUNA,14 pUSA15 and pAdeA16 in addition to pPTRI17 with the pyrithiamine-resistant marker (Figure 1).
Use of multiple vectors enabled us to produce simple natural products. However, production of more complex natural product requires a large number of genes. In addition, transformation and screening of the metabolite producing-transformant are time-consuming steps (2-4 weeks/gene). For the rapid reconstitution of the biosynthetic machinery in A. oryzae, we used the vectors pUARA2 and pUSA2 carrying tandemly arranged promoter/terminator set,18 which were provided by Prof. Gomi. They constructed those vectors based on the previously developed pTAex3 and pUSA vectors containing the argB and sC-selectable markers, respectively. The vectors utilize the PamyB promoter, so that all of the target genes introduced at the cloning site can be overexpressed in A. oryzae. Tandem transformation utilizing two plasmids (genes: A, C; plasmids: pA, pC) with the same selectable marker is efficient, requiring use of fewer vectors and allowing a rapid introduction of biosynthetic genes to obtain transformants (AO-A/C, AO-A, AO-C) (Figure 3). Analysis of these transformants provides sufficient data for the identification of individual gene functions (Figure 3). In the biosynthetic study of natural product, a rapid process is preferable for screening of the genes essential for the conversion of interest.
3. Indole diterpenes produced by various fungi
Indole-diterpenes (IDTs) were initially isolated from various ascomycetes fungi as potent tremorgenic mammalian mycotoxins. They also show additional unique biological activities such as inhibition of calcium-activated potassium channels,19 tremorgenic activity,20 potent and selective progesterone receptor agonistic activity,21 and anti-MRSA activity.22 Their wide range of biological activities are associated with their structural diversity. IDTs have various types of core structures which consist of indole and C20-polyprenyl moieties, and further modifications, such as prenylation and oxygenation (hydroxylation, epoxidation, and oxidative cyclization) yield more than one hundred family members.23,24 Repesentative IDTs include the simple emindole, nominine, and paspaline (1), and the more elaborated members paxilline (2), aflatrems (3), lolitrems, nodulisporic acid and penitrems (4) (Figure 4). Intriguingly, many of the fungi that form symbiotic relationships with plants produce these toxins, suggesting that the toxin-producing ability of the fungi may contribute to establish these ecological associations.
4. Enzymatic synthesis of fungal indole diterpenes
4-1. Paspaline
Nearly 70% of IDTs have a characteristic hexacyclic skeleton that is presumably derived from paspaline (1) (Figure 4). Therefore, elucidation of a detailed reaction pathway for the core scaffold is important for versatile enzymatic synthesis of IDTs. Previous feeding experiments using tryptophan or anthranilic acid25,26 suggested that either tryptophan or its precursors, such as indole-3-glycerol phosphate (IGP: 5), could be used as a substrate for the synthesis of a common intermediate, 3-geranylgeranyl indole (GGI: 6) (Schemes 2 and 3). A series of feeding experiments with 13C-labeled acetate revealed that the terpenoid core structure is derived from geranylgeranyl diphosphate (GGPP: 7).25,27 The structure of the less-modified indole diterpenes, paspaline suggested that 1 is a cyclization product of 6. This was experimentally supported by incorporation of deuterium labeled 6 into 1.28
In 2001, the first biosynthetic gene cluster for biosynthesis of an IDT, paxilline (pax) was identified from Penicillium paxilli.29 Gene inactivation and gene transfer to paxilline negative deletion mutants confirmed that four pax genes, paxG (GGPP synthase), paxC (prenyltransferase), paxM (FAD dependent monooxygenase), and paxB (hypothetical membrane protein), are required for the biosynthesis of 1.29,30 Recently, two more biosynthetic gene clusters for aflatrem (atm) and lolitrem (ltm) have been identified; four homologous genes (atmGCMB and ltmGCMB) were identified, adding to our understanding of the biosynthesis of indole diterpenes.31,32 Although paxB shows homology to a functionally characterized pyr4, a membrane binding protein catalyzing epoxide-opening initiated cyclization in meroterpenoid pyripyropene biosynthesis,33 little is known about the detailed function of the products of these four genes.
To confirm the roles of the four essential genes, we prepared four plasmids, pAdeA-paxG, pTAex3-paxC, pUSA-paxM, and pAdeA-paxB. Using the plasmids pAdeA-paxG and pTAex3-paxC, we transformed A. oryzae NSAR1 to obtain the transformant AO-paxGC, which produced 6 (7.7 mg/L). To this transformant, we further introduced paxM, yielding AO-paxGCM, which produced epoxy-GGI (8) (32 mg/L). Further incorporation of paxB into the transformant yielded AO-paxGCMB, which produces 1 (57 mg/L). The successful in vivo synthesis of 1 allowed us to determine the outline of the paspaline biosynthesis catalyzed by the four gene products (Scheme 2).34 Interestingly, unlike total synthesis of natural products, the yield of each product was increased as the number of incorporated genes increased. However, following the elucidation of the biosynthetic pathway, two questions remained: 1) determination of the actual substrates of the PaxC reaction; 2) the cyclization mechanism catalyzed by PaxM and PaxB.
To determine the substrate of the initial geranylgeranylation step in the GGI forming reaction, in collaboration with Prof. Dairi, we performed an in vitro analysis using recombinant PaxC, which has a characteristic diphosphate-binding motif, to yield reactive allylic carbocation for alkylation of the indole ring at the C3 position. As expected from the previous reports, 5 undergoes geranylgeranylation by PaxC to afford 6. Surprisingly, the reaction also proceeds using an indole as the substrate, although kinetic analysis showed that 5 is the preferred substrate for PaxC (kcat/KM for 5; 28.2 mM-1s-1, indole; 3.6 mM-1s-1). Further analysis of the reaction product revealed that PaxC catalyzes geranylgeranylation of 5, accompanied by elimination of glyceraldehyde 3-phosphate as a byproduct. This dearomatization-elimination process is novel in the reaction of prenyltransferases (Scheme 3). To the best of our knowledge, the only previously known example is an octaprenyltransferase, MenA, which catalyzes simultaneous prenylation and decarboxylation in menaquinone biosynthesis in Escherichia coli.35
We then turned our attention to the mechanism of the subsequent epoxidation and cyclization. Isolation of 8 and 1 from the AO-paxGCM and the AO-paxGCMB suggested that PaxM catalyzes regioselective epoxidation to give 8, and the subsequent cyclization of 8 yields the emindole SB (9).36 However, the reaction did not terminate at this stage and a second round of epoxidation/cyclization of 9 afforded 1 (Scheme 4). To validate this stepwise epoxidation/cyclization mechanism, we performed a biotransformation study using 8 and the bisepoxide 10, synthesized by the sequential Sharpless asymmetric dihydroxylations, as substrates. Incubation of 8 with a paxB-containing transformant, AO-paxB, of which was confirmed by semi-quantitative RT-PCR,29 led to the formation of 9. Importantly, AO-paxB also converted 10 into 1. These results showed a previously unknown mechanism involving two rounds of stepwise epoxidation-cyclization via 9 (Scheme 4). In the biosynthesis of the polyether antibiotic, lasalocid, the epoxidase Lsd18 catalyzes sequential epoxidations and the cyclase Lsd19 catalyzes cascade cyclization of the bisepoxide (Scheme 5).37 Although PaxM and PaxB catalyze similar epoxidation-cyclizations, the reaction sequence is quite different from those found in the biosynthesis of polyether antibiotic.
Among the various known epoxide opening-initiated polyene cyclizations, catalysis of squalene 2,3-epoxide by oxidosqualene cyclase (OSC) to a complex tetaracyclic ring system is one of the most well-known.38 Although the amino acid sequences of OSC and PaxB are quite different, both enzymes catalyze similar sequential cyclizations. In 2003, Matsuda and co-workers reported biomimetic synthesis of petromindole from a regioisomer of epoxyGGI with a triterpene cyclase of plant origin (Scheme 6).39 This enzymatic synthesis provided further support for the similarity between the reactions catalyzed by PaxB and steroid/triterpene cyclases. Biomimetic synthesis of protected epoxyGGI analogs with Lewis acids produced a multicyclic product similar to IDT in moderate yields.40 However, no product containing a paspaline-like ring system was produced.
4-2. Paxilline
In the paxilline (2) gene cluster described in Section 4-1, two genes encoding cytochrome P450 monooxygenases, paxP and paxQ, were identified. Their functions were examined by gene disruption studies of paxP and paxQ, which led to accumulation of paspaline (1) and 13-desoxypaxilline (11), respectively (Scheme 7). A feeding experiment using 1 and β-PC-M6 (12), conducted in a transformant harboring paxP showed their conversion to 11. These data indicated that PaxP and PaxQ catalyze multiple oxidation steps of 1 to 2 via 12 and 11.41
To achieve synthesis of 2 in A. oryzae, we added two more genes paxPQ to the AO-paxGCMB. Due to limitations of the available vectors, we used tandem transformation to introduce two genes with a single vector, pUNA. In this transformation, two types of transformants, one containing paxP and the other containing paxPQ, were obtained. The former, AO-paxGCMBP, produced 11 and the latter, AO-GCMBPQ, produced 2 (35 mg/L) (Scheme 7). 11, an oxidative product of 1, is constructed by oxidative elimination of a methyl group as formic acid, via 1) stepwise oxidation of a methyl group to aldehyde, and 2) abstraction of a neighboring hydrogen atom to eliminate HCO2H. Subsequent allylic oxidation of 11 gives 2.
In the cholesterol biosynthesis, an angular C14-methyl group is oxidatively eliminated to give olefin and formic acid.42 After hydroxylation and dehydrogenation catalyzed by cytochrome P450, the resultant formyl group is oxidatively eliminated to give olefin and formic acid (Scheme 7). In the reaction catalyzed by PaxP, a similar multistep oxidation proceeds to give olefin but the reaction is not terminated at this point. Subsequently, PaxP hydroxylates at C10 to give β-alcohol 12, that is further oxidized to the ketone 11. PaxQ hydoxylates 11 at the C13 position to afford 2. Similar multiple oxidations occur during the biosynthesis of isotrichotriol43,44 and gibberellins,45 suggesting that they are a common synthetic strategy in fungal metabolite biosynthesis.
4-3. Aflatrem
The potent mammalian tremorgens aflatrems (3), which are produced by A. flavus are structurally related to 2.46 The core skeleton of 3 is presumably derived by modifications of 2 (C20/C21 prenylation and C7 oxidation) as shown in Scheme 8. The structural similarity suggested that the aflatrem gene cluster would contain a gene set for construction of a paxilline skeleton. A conventional genomic DNA library search using a specific DNA probe and a bioinformatics-guided search of genome sequences has revealed aflatrem clusters (atm), which consists of the paspaline core construction genes (atmGCMB) and the oxidative modification genes (atmPQ) and an aflatrem-specific prenylation gene (atmD). Involvement of this cluster in aflatrem biosynthesis was supported by atmPQ gene complementation experiments of utilizing a paxPQ deletion mutant of a paxilline-producing strain, thus revealing the involvement of cytochrome P450s (AtmPQ) in the oxidative transformation of 1 to give paspalinine (13).31 Recently, the function of the prenyltransferase AtmD was studied by in vitro analysis utilizing 2 instead of 13.47
To develop a versatile method for synthesis of IDTs via 1, we examined the reconstitution of 1 in A. oryzae applying a tandem transformation method, which is a rapid and an efficient gene incorporation method to save the number of vectors and to shorten a length of time for reconstitution and functional analyses. The first tandem transformation of A. oryzae NSAR1 was carried out with pUARA2-paxGC and pUARA2-paxMB. One of the more than twenty transformants obtained from a single transformation produced 1 (54 mg/kg). A second tandem transformation of this transformant with pUSA2-atmPQ and pUSA-atmD gave three transformants producing 13 (88 mg/kg) and another three transformants producing aflatrem (3a) and β-aflatrem (3b) (54 mg/kg, 18 mg/kg). This is the first report of the total biosynthesis of 3 (Scheme 8). Remarkably, the introduction of the 7 genes required for aflatrem biosynthesis was achieved in only two rounds of transformations.18
PaxQ homologs usually catalyze only C13-hydroxylation whereas AtmQ catalyzes oxidative cyclization at C7 and C13-hydroxylation to yield 13, although we could not isolate a putative intermediate, paspalicine.48 The reaction commenced with oxidation at the C7 allylic position and the resultant hemiacetal gave a cyclic acetal with a hydroxyl group at C27 to give paspalicine. Subsequent oxidation at the C13 allylic position of paspalicine yielded 13 (Scheme 9).
Dimethylallyl transferase (DMAT) is a well-known modification enzyme that is used in the biosynthesis of various natural products. Prenylation commonly occurs alcohol and amino groups. In addition, C-prenylation of the aromatic substrates such as indole moiety of IDTs is also known in the late stage of biosynthesis.49,50 To date, the known DMATs involving IDT biosynthesis catalyze highly regioselective C-prenylation at C2, and C4-C7 positions of indole. The prenylation modes (α vs γ) are strictly controlled by individual DMATs. In the aflatrem biosynthesis, AtmD showed γ-selective prenylation although the regioisomers 3a and 3b were obtained in a 3:1 ratio (Scheme 9).
Very recently, Tang and co-workers reported combinatorial synthesis of IDTs in A. flavus. This fungus contains a stand-alone cyclase, AfB (an atmB homolog).51 Co-expression of AfB with atmGCM affords the alternative IDTs nominine and aflavinine, along with aflatrems. This observation indicates that after dupulication of IDT cluster, A. flavus chose combinatorial synthesis (keeping a single copy of the gene encoding cyclization enzyme) over independent synthesis of IDTs using two individual clusters.
4-3. Penitrem
To apply the simple and rapid reconstitution method to the elucidation of the synthesis of the most highly elaborated natural products, we chose the indole diterpene, penitrems (4), which has a unique tricyclic system adjacent to the paxilline core structure (Figure 4). Biosynthetic studies with isotopically labeled precursors including paxilline, suggested that the characteristic penitrem core scaffold is derived from a diprenylated paxilline derivative.25,52
At the beginning, structural analysis of penitrem congeners A~F isolated from P. crustosum53,54 suggested that the late 3-step conversions of penitrem D (4d) to A (4a) consists of epoxidation (4d to B (4b)), benzylic hydroxylation (4b to E (4e)) and promiscuous chlorination (4d to C (4c); 4b to F (4f); 4e to 4a). In addition, based on isolation of potential intermediates, paspaline (1),55 PC-M4 (14), and PC-M5 (15),56,57 we hypothesized that its biosynthetic pathway was divided into 3 stages (Scheme 10); stage I: from IGP (5) and GGPP (7) to paxilline (2) or its equivalent via 1; stage II: 2 to 14 via 15; stage III: 14 to 4a. At this point, we can not eliminate the possibility that they are dead end products from biosynthetic intermediates.
We then performed a genomic analysis of the penitrem-producing fungus P. simplicissimum. Using gene cluster search tools (a local BLAST search and the 2ndFind program58), we identified a gene cluster that contained genes homologous to those of the paxilline gene cluster. However, this cluster lacked several genes for oxidative modifications of the indole ring. Therefore, we performed an RNA-seq analysis of another penitrem producer, P. crustosum. This analysis identified a second gene cluster, and the expression profile of these genes was closely correlated to that of the genes in cluster I. Eventually, we identified a penitrem gene cluster (ptm) consisting of genes encoding 17 biosynthetic enzymes (6 genes homologous to the paxilline biosynthetic genes, ptmGCMBPQ; 2 prenyltransferase genes, ptmDE; 6 genes encoding oxidative transformation enzymes, ptmJKLNOU; and 3 genes for modification enzymes, ptmHIV.
Initially, we examined the Stage I transformation. As in the case of aflatrem, we introduced the ptmPQ genes to the transformant AO-paxGCMB, which produces 1. This transformant produced 2 (8.8 mg/kg) but not β-PC-M6 (12), suggesting that PtmP catalyzes a 5-step transformation, similar to PaxP, and that the functionally unknown enzyme reduces 2 to a β-alcohol (Scheme 11).
During Stage II, two prenylations, a C10 reduction and a dehydration, are expected to occur. To determine the biosynthetic reaction sequence, we prepared the transformant AO-ptmHD by introducing the ptmHD genes, which encode a NAD(P+)-dependent oxidoreductase and a prenyltransferase, into A. oryzae NSAR1. The resultant transformant converted 2 to β-paxitriol (16) and a C20-prenylated analog (17) of 16 (Schemes 11, 12). Considering the remaining transformations in the Stage II, 3 genes (ptmE, prenyltransferase; ptmV, acetyltransferase; ptmI, unknown) are likely involved in the reaction. Three transformants, AO-ptmHDV, AO-ptmHDVI and AO-ptmHDVIE, were prepared from AO-ptmHD. While incubation of AO-ptmHDV with 2 did not give a predominant product, that of AO-ptmHDVI gave the 20-prenylpenijanthine (19) (Scheme 11). Although no acetylation product was obtained, this formal dehydration process likely involves acetylation and elimination of acetic acid. Similar formal dehydration processes (acetylation and elimination) have been reported in the biosynthesis of tetronate antibiotics.59,60
To obtain additional information on the reaction sequence involved in Stage II, we employed an in vitro functional analysis of the prenyltransferase PtmD. Enzymatic reactions with three substrates, 2, 16 and penijanthine (18), synthesized from paxilline with the recombinant PtmD in the presence of dimethylallyl diphosphate (DMAPP) showed a clear substrate preference, in the following order: 16, 18, and 2 (Scheme 12). This result suggested that the three-step conversion proceeded from C10-ketoreduction (PtmH), to C20-prenylation (PtmD), to dehydration (PtmV, PtmI). In the microbial conversion of 2 with AO-ptmHDVIE, no product other than 19 was obtained, indicating that, prior to PtmE catalyzed prenylation, an oxidation is involved in the later step of Stage II (most likely after the second prenylation). Thus, we turned our attention to functional analysis of the genes encoding oxidation enzymes, to reduce the number of possible reaction pathways (Scheme 12).
To address the critical issue of whether PC-M4 (14) is a shunt metabolite or an actual intermediate, we prepared transformants AO-ptmKLJ, AO-ptmKUN, AO-ptmKULNJ and AO-ptmKULNO. Microbial conversion of 14 with AO- ptmKLJ or AO-ptmKUN gave a new product secopenitrem D (20) and 4c, respectively. In addition, AO-ptmKULNJ and AO-ptmKULNO afforded 4a and 4f, respectively. These results showed that 14 is an intermediate of penitrem biosynthesis, and also that the five genes ptmKULNJ are essential for the Stage III transformations, and that PtmJ catalyzes the last benzylic hydroxylation (Scheme 13). To elucidate the detailed biosynthetic pathway of the Stage III transformations, we prepared the transformants AO-ptmL, AO-ptmK, AO-ptmN and AO-ptmJ. The conversion of 14 with these transformants established that the reaction proceeds via a 5-step sequential oxidative transformation (Scheme 14); the first half involves ring expansion-cyclic ether formation and the latter half involves epoxidation-hydroxylation-chlorination.
To elucidate the skeletal construction of 14, we introduced two additional genes, ptmOE, into AO-ptmHDVI. Incubation of 2 with the resultant AO-ptmHDVIOE yielded 14. The in vitro transformation of 19 with the recombinant flavin-dependent monooxygenase PtmO yielded a single acid-labile product, PC-M5 (15). Incubation of 15 with the recombinant PtmE in the presence of Mg2+ and DMAPP gave 14. PtmE did not accept any other precursors. These data established that the Stage II biosynthetic pathway proceeds from 2 to 14 via the key intermediates 19 and 15 (Scheme 15).
In overall enzymatic transformations, the construction mechanism of bicyclo[4,2,0]octane system is especially intriguing. Prenylation-initiated cationic cyclizations are rather rare transformation in natural product biosynthesis (Schemes 15, 16). Head-to-head couplings of DMAPP and the subsequent cyclization give chrysanthemyl diphosphate (cyclopropane)61 and cyclolavandulyl diphosphate (cyclohexene).62 A bicyclo[3,3,1]nonane system of the antidepressant agent hyperforin was proposed to be constructed by prenylation of the geranyl group at the phloroglucinol moiety, followed by the nucleophilic attack of the internal enol.63,64 The oxidative ring expansion catalyzed by a cytochrome P450 monooxygenase PtmK is another highlight of the penitrem biosynthesis (Scheme 16), although a similar ring expansion catalyzed by a non-heme Fe/α-ketoglutarate dependent dioxygenase has been reported in the biosynthesis of cephalosporin.65 Subsequent formation of the 8-membered oxocane cyclic ether is catalyzed by the cytochrome P450 monooxygenase PtmU (Scheme 16). In organic synthesis, this type of medium-sized ring formation is rather difficult to achieve owing to non-bonding transannular repulsion. A previous example of oxidative cyclic ether formation catalyzed by a cytochrome P450 has been reported in the biosynthesis of aureothin.66
5. Conclusion
It has long been believed that biosynthesis of natural products is a black box, and that the reactions could not be reproduced in laboratories. However, recent remarkable progress in DNA sequencing and bioinformatic technologies has enabled us to obtain blueprints (in the form of a list of biosynthetic genes) for natural products. With this information, elucidation of all of the individual enzymatic reaction steps, using an appropriate expression system, provides sufficient knowledge of how complex molecules are synthesized in nature. Once we can elucidate the detailed biosynthetic mechanism of a representative member of a specific family of natural products, we can propose a versatile plan for synthesis of family members, as in organic synthesis.
In this review, we describe our approach for synthesis of fungal indole diterpenes. Using the robust A. oryzae expression system, we developed a general strategy for constructing the core scaffold using 3 reaction steps (introducing an oligoprenyl group to the indole; regiospecific epoxidation; and programmed cyclization). Based on this finding, we developed a rapid method for construction of a transformant harboring the key genes paxGCMB, which could produce the versatile precursor paspaline. Using this transformant, we synthesized a simple paxilline (2 genes), aflatrems (3 genes), and a highly elaborated decacyclic penitrem (2 + 6 + 5 genes). This powerful approach enables us not only to synthesize a target natural product but also to access intermediates which are not readily synthesized. Thus, we are able to study intriguing reaction mechanisms, such as the construction of the bicyclo[4.2.0]octane core scaffold via the bicyclo[3.2.0]heptane system, in the biosynthesis of penitrem. Through this project, we showed that the A. oryzae heterologous expression system is highly reliable for studying fungal natural product biosynthesis.
Because biosynthetic approaches typically use enzymes whose catalytic efficiencies have already been optimized, we used the catalyst without further modification. Without optimizing the expression level, we were able to produce the target molecule at a level of ~100 mg/L solely by screening for higher-yielding strains. In 2014, Sanofi produced artemisinic acid at 25 g/L using engineered yeast cells harboring a sesquiterpene synthase, a monooxygenase, and genes for 3 other enzymes.67 The intermediate acid is further converted to the anti-malarial agent artemisinin via a chemical process at a scale of 60 t/year. This example shows that industrial optimization can provide various improvements, such as increased substrate supply, shutout of branched pathways, and fermentation conditions sufficient for industrial production. Therefore, simple lab-scale production is a promising first step to be scaled up to an industrial level.
From a synthetic point of view, enzymatic regio- and stereoselective oxidations are unique for manipulation of highly functionalized intermediates without protecting groups. For example, more than half of the 17 enzymes in penitrem biosynthesis catalyze oxidative transformations such as hydroxylation, epoxidation, halogenation, oxidative elimination, oxidative ring expansion, and cyclic ether formation. In addition, recent studies have shown that multistep oxidative transformation with a single enzyme is frequently involved in the biosynthesis of natural products, as in the case of PaxP (5 reaction steps). Similar observations have been reported in the biosynthesis of the fungal metabolites T-2 toxin,43,44 austinol,68 terretonin,8 and fumagillin,69 representative enzymes for which include -ketoglutarate-dependent dioxygenases, monooxygenases of cytochrome P450, and flavin-dependent enzymes. Modification of a small number of enzyme groups is sufficient to diversify natural products, suggesting that rational engineering of these oxidation enzymes can be applied for oxidations of both basic and applied chemical process.
Currently, a number of oxidation enzymes have been characterized. Thus, in the near future we can expect to construct a huge library of useful enzymes. Considering that stereostructures can be experimentally determined or obtained by precise simulation, we may be able to create the desired catalysts by rational design and engineering of enzymes and altering their substrate specificity.
ACKNOWLEDGEMENTS
Authors sincerely thank to all co-workers and collaborators appeared in the original papers. This work was supported by the MEXT research grant on innovative area 22108002 to H. Oikawa.
References
1. R. Robinson, J. Chem. Soc., Trans., 1917, 111, 762. CrossRef
2. T. Ugai, A. Minami, R. Fujii, M. Tanaka, H. Oguri, K. Gomi, and H. Oikawa, Chem. Commun., 2015, 51, 1878. CrossRef
3. R. Fujii, T. Ugai, H. Ichinose, M. Hatakeyama, T. Kosaki, K. Gomi, I. Fujii, A. Minami, and H. Oikawa, Biosci. Biotechnol. Biochem., in press, DOI: 10.1080/09168451.2015.1104234. CrossRef
4. R. Fujii, A. Minami, T. Tsukagoshi, N. Sato, T. Sahara, S. Ohgiya, K. Gomi, and H. Oikawa, Biosci. Biotechnol. Biochem., 2011, 75, 1813. CrossRef
5. R. Chiba, A. Minami, K. Gomi, and H. Oikawa, Org. Lett., 2013, 15, 594. CrossRef
6. Y. Ye, A. Minami, A. Mandi, C. Liu, T. Taniguchi, T. Kuzuyama, K. Monde, K. Gomi, and H. Oikawa, J. Am. Chem. Soc., 2015, 137, 11846. CrossRef
7. Y. Matsuda, T. Wakimoto, T. Mori, T. Awakawa, and I. Abe, J. Am. Chem. Soc., 2014, 136, 15326. CrossRef
8. Y. Matsuda, T. Iwabuchi, T. Wakimoto, T. Awakawa, and I. Abe, J. Am. Chem. Soc., 2015, 137, 3393. CrossRef
9. P. Barbesgaard, H. P. Heldt-Hansen, and B. Diderichsen, Appl. Microbiol. Biotechnol., 1992, 36, 569. CrossRef
10. D. Lubertozzi and J. D. Keasling, Biotechnol. Adv., 2009, 27, 53. CrossRef
11. S. Tada, Y. Iimura, K. Gomi, K. Takahashi, S. Hara, and K. Yoshizawa, Agric. Biol. Chem., 1989, 53, 593. CrossRef
12. (a) T. Fujii, H. Yamaoka, K. Gomi, K. Kitamoto, and C. Kumagai, Biosci. Biotechnol. Biochem., 1995, 59, 1869; CrossRef (b) K.-X. Huang, I. Fujii, T. Ebizuka, K. Gomi, and U. Sankawa, J. Biol. Chem., 1995, 270, 21495. CrossRef
13. F. J. Jin, J. Maruyama, P. R. Juvvadi, M. Arioka, and K. Kitamoto, FEMS Microbiol. Lett., 2004, 239, 79. CrossRef
14. O. Yamada, B. R. Lee, K. Gomi, and Y. Iimura, J. Biosci. Bioeng., 1999, 87, 424. CrossRef
15. O. Yamada, S. N. Nan, T. Akao, M. Tominaga, H. Watanabe, T. Satoh, H. Enei, and O. Akita, J. Biosci. Bioeng., 2003, 95, 82. CrossRef
16. F. J. Jin, J. Maruyama, P. R. Juvvadi, M. Arioka, and K. Kitamoto, Biosci. Biotechnol. Biochem., 2004, 68, 656. CrossRef
17. T. Kubodera, N. Yamashita, and A. Nishimura, Biosci. Biotechnol. Biochem., 2002, 66, 404. CrossRef
18. K. Tagami, A. Minami, R. Fujii, C. Liu, M. Tanaka, K. Gomi, T. Dairi, and H. Oikawa, ChemBioChem, 2014, 15, 2076. CrossRef
19. H.-G. Knaus, O. B. McManus, S. H. Lee, W. A. Schmalhofer, M. Garcia-Calvo, L. M. H. Helms, M. Sanchez, K. Giangiacomo, and J. P. Reuben, Biochemistry, 1994, 33, 5819. CrossRef
20. C. O. Miles, A. L. Wilkins, R. T. Gallagher, A. D. Hawkes, S. C. Munday, and N. R. Towers, J. Agric. Food Chem., 1992, 40, 234. CrossRef
21. D. M. Roll, L. R. Barbieri, R. Bigelis, L. A. McDonald, D. A. Arias, L.-P. Chang, M. P. Singh, S. W. Luckman, T. J. Berrodin, and M. R. Yudt, J. Nat. Prod., 2009, 72, 1944. CrossRef
22. M. Ogata, J. Ueda, M. Hoshi, J. Hashimoto, T. Nakashima, K. Anzai, M. Takagi, and K. Shinya, J. Antibiot., 2007, 60, 645. CrossRef
23. 'Handbook of Secondary Fungal Metabolites, 3-Volume Set', ed. by R. J. Cole, B. B. Jarvis, and M. A. Schweikert, Elsevier, 2003.
24. 'Fungal Metabolites', Vol. 2, ed. by W. B. Turner, Academic Press Inc., 1982.
25. A. E. de Jesus, C. P. Gorst-Allman, P. S. Steyn, F. R. van Heerden, R. Vleggaar, P. L. Wessels, and W. E. Hull, J. Chem. Soc., Perkin Trans. 1, 1983, 1863.
26. K. M. Byrne, S. K. Smith, and J. G. Ondeyka, J. Am. Chem. Soc., 2002, 124, 7055. CrossRef
27. W. Acklin, F. Weibel, and D. Arigoni, Chimia, 1977, 31, 63.
28. S. Fueki, T. Tokiwano, H. Toshima, and H. Oikawa, Org. Lett., 2004, 6, 2697. CrossRef
29. C. Young, L. McMillan, E. Telfer, and B. Scott, Mol. Microbiol., 2001, 39, 754. CrossRef
30. S. Saikia, E. J. Parker, A. Koulman, and B. Scott, FEBS Lett., 2006, 580, 1625. CrossRef
31. M. J. Nicholson, A. Koulman, B. J. Monahan, B. L. Pritchard, G. A. Payne, and B. Scott, Appl. Environ. Microbiol., 2009, 75, 7469. CrossRef
32. C. A. Young, M. K. Bryant, M. J. Christensen, B. A. Tapper, G. T. Bryan, and B. Scott, Mol. Gen. Genomics, 2005, 274, 13. CrossRef
33. T. Itoh, K. Tokunaga, Y. Matsuda, I. Fujii, I. Abe, Y. Ebizuka, and T. Kushiro, Nat. Chem., 2010, 2, 858. CrossRef
34. K. Tagami, C. Liu, A. Minami, M. Noike, T. Isaka, S. Fueki, Y. Shichijo, H. Toshima, K. Gomi, T. Dairi, and H. Oikawa, J. Am. Chem. Soc., 2013, 135, 1260. CrossRef
35. K. Suvarna, D. Stevenson, R. Meganathan, and M. E. S. Hudspeth, J. Bacteriol., 1998, 180, 2782.
36. K. Nozawa, M. Yuyama, S. Nakajima, K. Kawai, and S. Udagawa, J. Chem. Soc., Perkin Trans. 1, 1988, 2155. CrossRef
37. (a) A. Minami, H. Oguri, K. Watanabe, and H. Oikawa, Curr. Opin. Chem. Biol., 2013, 17, 555; CrossRef (b) A. Minami, M. Shimaya, G. Suzuki, A. Migita, S. S. Shinde, K. Sato, K. Watanabe, T. Tamura, H. Oguri, and H. Oikawa, J. Am. Chem. Soc., 2012, 134, 7246; CrossRef (c) K. Hotta, X. Chen, R. S. Paton, A. Minami, H. Li, K. Swaminathan, I. Mathews, K. Watanabe, H. Oikawa, K. N. Houk, and C.-Y. Kim, Nature, 2012, 483, 355. CrossRef
38. K. Ulrich Wendt, G. E. Schulz, E. J. Corey, and D. R. Liu, Angew. Chem. Int. Ed., 2000, 39, 2812. CrossRef
39. Q. Xiong, X. Zhu, W. K. Wilson, A. Ganesan, and S. P. T. Matsuda, J. Am. Chem. Soc., 2003, 125, 9002. CrossRef
40. (a) J. D. Rainier and A. B. Smith III, Tetrahedron Lett., 2000, 41, 9419; CrossRef (b) J. S. Clark, J. Myatt, C. Wilson, L. Roberts, and N. Walshe, Chem. Commun., 2003, 1546. CrossRef
41. S. Saikia, E. J. Parker, A. Koulman, and B. Scott, J. Biol. Chem., 2007, 282, 16829. CrossRef
42. G. I. Lepesheva, and M. R. Waterman, Biochim. Biophys. Acta, 2007, 1770, 467.
43. M. Kimura, T. Tokai, N. Takahashi-Ando, S. Ohsato, and M. Fujimura, Biosci. Biotechnol. Biochem., 2007, 71, 2105. CrossRef
44. T. Tokai, H. Koshino, N. Takahashi-Ando, S. Ohsato, M. Fujimura, and M. Kimura, Biochem. Biophys. Res. Commun., 2007, 353, 412. CrossRef
45. C. A. Citron, N. L. Brock, B. Tudzynski, and J. S. Dickschat, Chem. Commun., 2014, 50, 5224. CrossRef
46. R. T. Gallagher, J. Clardy, and B. J. Wilson, Tetrahedron Lett., 1980, 21, 239. CrossRef
47. C. Liu, A. Minami, M. Noike, H. Toshima, H. Oikawa, and T. Dairi, Appl. Environ. Microbiol., 2013, 79, 7298. CrossRef
48. T. H. Fehr and W. Acklin, Helv. Chim. Acta, 1966, 49, 1907. CrossRef
49. S.-M. Li, Nat. Prod. Rep., 2010, 27, 57. CrossRef
50. T. Bonits, V. Alva, O. Saleh, A. N. Lupas, and L. Heide, PLoS One, 2011, 6, e27336. CrossRef
51. M.-C. Tang, H.-C. Lin, D. Li, Y. Zou, J. Li, W. Xu, R. A. Cacho, M. E. Hillenmeyer, N. K. Garg, and Y. Tang, J. Am. Chem. Soc., 2015, 137, 13724. CrossRef
52. J. Penn and P. G. Mantle, Phytochemistry, 1994, 35, 921. CrossRef
53. A. E. de Jesus, P. S. Steyn, F. R. van Heerden, R. Vleggaar, P. L. Wessels, and W. E. Hull, J. Chem. Soc., Perkin Trans. 1, 1983, 1847. CrossRef
54. A. E. de Jesus, P. S. Steyn, F. R. van Heerden, R. Vleggaar, P. L. Wessels, and W. E. Hull, J. Chem. Soc., Perkin Trans. 1, 1983, 1857.
55. M. C. González, C. Lull, P. Moya, I. Ayala, J. Primo, and E. Primo Yúfera, J. Agric. Food Chem., 2003, 51, 2156. CrossRef
56. T. Hosoe, K. Nozawa, S. Udagawa, S. Nakajima, and K. Kawai, Chem. Pharm. Bull., 1990, 38, 3473. CrossRef
57. T. Yamaguchi, K. Nozawa, T. Hosoe, S. Nakajima, and K. Kawai, Phytochemistry, 1993, 32, 1177. CrossRef
58. 2ndFind: http ://biosyn.nih.go.jp/2ndfind/.
59. C. Kanchanabanca, W. Tao, H. Hong, Y. Liu, F. Hahn, M. Samborskyy, Z. Deng, Y. Sun, and P. F. Leadlay, Angew. Chem. Int. Ed., 2013, 52, 5785. CrossRef
60. L.-F. Wei, H.-Y. He, H.-X. Pan, L. Han, R. Wang, and G.-L. Tang, Org. Lett., 2014, 16, 1578. CrossRef
61. S. B. Rivera, B. D. Swedlund, G. J. King, R. N. Bell, C. E. Hussey, Jr., D. M. Shattuck-Eidens, W. M. Wrobel, G. D. Peiser, and C. D. Poulter, Proc. Natl. Acad. Sci. U.S.A., 2001, 98, 4373. CrossRef
62. T. Ozaki, P. Zhao, T. Shinada, M. Nishiyama, and T. Kuzuyama, J. Am. Chem. Soc., 2014, 136, 4837. CrossRef
63. P. Adam, D. Arigoni, A. Bacher, and W. Eisenreich, J. Med. Chem., 2002, 45, 4786. CrossRef
64. N. S. Bystrov, B. K. Chernov, V. N. Dobrynin, and M. N. Kolosov, Tetrahedron Lett., 1975, 16, 2791. CrossRef
65. (a) K. Valegård, A. C. T. van Scheltinga, A. Dubus, G. Ranghino, L. M. Öster, J. Hajdu, and I. Andersson, Nat. Struct. Mol. Biol., 2004, 11, 95; CrossRef (b) K. Valegard, A. C. T. van Scheltinga, M. D. Lloyd, T. Hara, S. Ramaswamy, A. Perrakis, A. Thompson, H. J. Lee, J. E. Baldwin, C. J. Schofield, J. Hajdu, and I. Andersson, Nature, 1998, 394, 805. CrossRef
66. M. E. A. Richter, N. Traitcheva, U. Knüpfer, and C. Hertweck, Angew. Chem. Int. Ed., 2008, 120, 9004. CrossRef
67. A. Bauer and M. Brönstrup, Nat. Prod. Rep., 2014, 31, 35. CrossRef
68. Y. Matsuda, T. Awakawa, T. Wakimoto, and I. Abe, J. Am. Chem. Soc., 2013, 135, 10962. CrossRef
69. H.-C. Lin, Y. Tsunematsu, S. Dhingra, W. Xu, M. Fukutomi, Y.-H. Chooi, D. E. Cane, A. M. Calvo, K. Watanabe, and Y. Tang, J. Am. Chem. Soc., 2014, 136, 4426. CrossRef