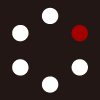
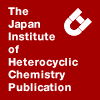
HETEROCYCLES
An International Journal for Reviews and Communications in Heterocyclic ChemistryWeb Edition ISSN: 1881-0942
Published online by The Japan Institute of Heterocyclic Chemistry
e-Journal
Full Text HTML
Received, 17th June, 2016, Accepted, 9th August, 2016, Published online, 18th August, 2016.
■ Diazonaphthoquinones: Synthesis, Reactions and Applications
Dina I. A. Othman and Mitsuru Kitamura*
Department of Applied Chemistry, Faculty of Engineering, Kyushu Institute of Technology, 1-1 Sensuicho, Tobata, Kitakyushu, 804-8550, Japan
Abstract
This review deals with synthesis and reactions of diazonaphthoquinones during the past 6 years. Various substituted diazonaphthoquinones have been prepared by the reaction of the appropriate naphthol and 2-azido-1,3 dimethyl imidazolinium chloride (ADMC) in short direct pathway. This method is likely to find wide spread uses in organic synthesis and material chemistry such as for preparing photoresists. In this review, different metal catalyzed reactions are investigated and applied successfully to the synthesis of important aromatic compounds. In addition, total synthesis of some natural compounds has been attempted using this recently developed diazo transfer methodology. It is hoped that this compilation, in combination with the previously published literatures on diazo-transfer reaction, will provide useful, up-to-date and comprehensive foundation and reference sources for individuals interested in the same field.CONTENTS
1. Introduction
2. Synthetic method
3. Application of diazo-transfer reaction
3-1. Metal-catalyzed reaction
3-2. Synthetic study of natural products
4. Conclusion
1. INTRODUCTION
Recently, orthodiazonaphthoquinones [1-diazo-2(1H)-naphthalenones (1) and 2-diazo-1(2H)-naphthalenones (2)], have been the subject of much interest because of their unique structures, reactivity and few known preparative method (Figure1).1 They are unique cyclic α-diazocarbonyl compounds, which are used as building blocks candidates for many aromatic functional materials, such as solar cells, metal ligands and antioxidants.2,3 The Wolff rearrangement of 1,2-diazonaphthoquinones is the key reaction of photoresists, therefore they are exclusively used as photoresist materials, such as Novolak–diazonaphthoquinone resists.4-6 However, the development of diverse reactions has been partially limited due to their difficult synthesis.1
Generally, diazonaphthoquinones are synthesized from naphthols using different pathways, either by diazotization of aminophenol derivatives followed by deprotonation (Route a)7 or by monosulfonylhydrazonation of quinones followed by elimination of sulfinic acid (Route b),8 as illustrated in Scheme 1. Multi-step reaction and the requirement of regioselective transformation were the main drawbacks of both routes, thus diazo-transfer reaction (Route c) was found to be the shortest direct synthetic method of diazonaphthoquinones although it is not so popular one.9
In 1978, Balli et al. have synthesized 2-azido-3-ethylbenzothiazolium tetrafluoroborate, as an efficient reagent for diazo-transfer reaction to naphthol, but this method suffered from low yield (eq. 1).10 Thus, the development of short efficient direct method for the synthesis of diazonaphthoquinones has become an urgent need.
Recently, we found that 2-azido-1,3-dimethylimidazolinium salts act as efficient and safe diazo-transfer reagents giving easily isolated and high yielded diazo products.11-15 2-Azido-1,3-dimethylimidazolinium chloride (ADMC) (3) could be used for the diazo-transfer reaction to naphthols to give corresponding various diazonaphthoquinones in good yield. Furthermore, we developed a new synthetic method of substituted naphthols by the metal-catalyzed reaction of diazonaphthoquinones, which could be applied for the synthetic study of natural products. In this review, we describe our efforts on the synthesis and application toward development of synthetic method of diazonaphthoquinones.
2. SYNTHETIC METHOD
Synthesis of diazonaphthoquinones by diazo-transfer reaction of reaction 2-azido-1, 3-dimethylimidazolinium chloride (ADMC) (3) with naphthols
Initially, the reaction of 1-naphthol with ADMC 3 was examined. To a solution of ADMC 3, prepared by reaction of chloroimidazolinium chloride (DMC) with sodium azide, 1-naphthol and triethylamine were added using acetonitrile at -20 C, as shown in Scheme 2. It is worth noting that; using 15-crown ether for such reaction was proved to be very effective in decreasing the reaction time and giving more clean reaction mixture. Protic solvent was not to be preferred, because diazonaphthoquinone products decomposed to naphthol in an aqueous solution. The choice of base was also important in this diazo-transfer reaction. Using Et3N, i-Pr2NEt and K2CO3 led to good yield. In contrast, using less basic bases, such as aromatic bases (imidazole, pyridine, 4-dimethylaminopyridine), were not suitable for the production of diazonaphthoquinone and using DBU (1,8-diazobicyclo[5.4.0]undec-7-ene) resulted in unknown products.
In Table 1, the results of the diazo-transfer reaction for various naphthols and related compounds are shown. 1-Nonsubstituted 2-naphthols were converted to 1-diazotized compounds in good yields (entries 1-5); while 1-substituted 2-naphthols did not yield corresponding diazo compounds (entries 6, 7). 2-Nonsubstituted 1-naphthol gave 2-diazo derivatives in good yields without influence of the substituent on C(3) or C(4) on naphthol (entries 8-15). The reaction of 2-alkyl-1-naphthol became complex and 4-diazotized compound was formed in low yield (entry 16). Anthrone (equivalent to anthracen-9-ol) gave the corresponding diazo compound (4) in good yield (entry 17), but phenol could not be used in the diazotization reaction (entry 18).
Interestingly, 1-formyl-2-naphthol gave isoxazole derivative as a major product, which is further transformed to nitrile under stirring for 4 h at rt, as shown in Scheme 3.
The results of the reaction for naphthalenediols are shown in Table 2. The formation of mono diazotized compounds was observed in most cases except for 1,3-naphthalenediol which gave the bis diazotized compound in 80% yield when 3 equiv. of 3 was used (entry 1). Subsequent acetylation of the monodiazotized products was performed so as to facilitate its isolation.
For the importance of diazo compounds in both semiconductor manufacturing and medicinal chemistry and in order to expand the applicability of this newly developed diazo-transfer methodology, the behavior of another OH group-containing heterocyclic scaffolds was explored as shown in Table 3.16 Some derivatives of quinoline, isoquinoline, indole and 4-hydroxycoumarin were transformed to corresponding diazotized compounds in moderate to good yield.
A plausible mechanism for the reaction between ADMC 3 and 2-naphthol in the presence of a base is shown in Scheme 4. The reaction pathway depends on the base used. Naphtholate I will be formed from 2-naphthol and the base at equilibrium when the used base has a conjugate acid with an acidity constant similar to that of naphthol (pKa 10). Naphtholate I will then attack the terminal nitrogen in 3 (the position a in 3) to form intermediate II. Intermediate II will then undergo intramolecular proton abstraction to afford the corresponding diazo compound 1 and guanidine III. The reverse reaction will occur when naphtholate I attacks the central carbon in the guanidinium (the position b in 3), and naphtholate I and 2-naphthol will be reformed at equilibrium. In contrast, naphtholate I will be kinetically formed when a strong base (pKaH > 10) is used, and a hard oxygen nucleophile will attack the most positive position b in 3 to form IV. In this case, the reverse reaction will be slow because naphtholate I is predominantly formed from 2-naphthol. Naphtholate I will hardly be formed when a weak base is used; therefore diazonaphthoquinone 1 will not be formed efficiently.
3. APPLICATIONS OF DIAZO-TRANSFER REACTION
The recently developed reaction was involved in many attractive chemical applications, either in different metal-catalyzed reaction with the aim to get efficient aromatic compounds or in total synthesis of natural compounds.
3-1. METAL-CATAYZED REACTION
Pd-Catalyzed formal OH insertion reaction of acetic acid
Similar to catechol derivatives, 1,2-naphthalenediols are very attractive candidates for aromatic functional materials, such as solar cells, metal ligands, and antioxidants.3,17 However, to date, few useful processes have been reported for their synthesis.18 In 2011, the synthesis of 1,2-naphthalenediol derivatives was first achieved via Pd(II)-catalyzed formal OH insertion reaction of 1,2-diazonaphthoquinones to acetic acid (eq. 2).19
Several reaction conditions were examined to obtain naphthalenediol monoacetate, which was then subjected to hydrolysis to yield the desired naphthalene diol. It was found that, rhodium(II) acetate, cupper salts (CuI, CuCl, CuCl2, Cu(OAc)2), or PdCl2 were ineffective while palladium(II) acetate was found to be the most efficient catalyst for the OH insertion reaction of diazonaphthoquinone. Then, synthesis of different 1,2-naphthalenediol derivatives from various diazonaphthoquinones was attempted (Table 4). It was observed that, the reactions of 1-diazonaphthoquinones required slightly higher temperature than those of 2-diazonaphthoquinones. Furthermore, it was found that the Pd(II)-catalyzed reaction performed in the presence of lithium halides produced halonaphthols comparable to the Sandmeyer reaction, as shown in eq. 3. In similar manner, Pd(OAc)2-catalyzed OH insertion reaction of typical acyclic -diazocarbonyl compounds was proved to yield acetoxy dicarbonyl compounds smoothly.
Rh-Catalyzed reaction with acetic anhydride
Exclusively, Scheme 5 illustrates the practical and efficient Rh2(OAc)4-catalyzed reaction of 1,2-diazo- naphthoquinones with acetic anhydride to form 1,2-naphthalenediol diacetates. Thus, protected 1,2-naphthalenediol derivatives could be synthesized and isolated efficiently. In this reaction, cupper salts and Pd catalyst were found to be inefficient while in the presence of Rh(II) acetate, diacetate products were obtained smoothly in good yield.20 The possible reaction mechanism for the formation of naphthalenediol diacetates is illustrated in Scheme 5.
The scope and limitations of the Rh2(OAc)4-catalyzed formation of 1,2-naphthalenediol diacetates from 1,2-diazonaphthoquinones are shown in Table 5. In all experiments, pyridine should be added to the reaction mixture following the reaction with catalytic Rh2(OAc)4 in acetic anhydride.
In addition, the Rh-catalyzed reaction of 2,4-bis(diazo)-1,2,3,4-tetrahydronaphthalene-1,3-dione (9) was examined to yield 1,2,3,4-tetra(acetoxy)naphthalene (10) in moderate yield (eq. 4).
Pd-Catalyzed cross coupling reaction with arylboronic acid
Introduction of substituents to aromatic compounds is a very important process in organic synthesis. Regioselective arylation of 1-naphthol remains one of the most difficult issues in the synthesis of the substituted phenol derivatives, so that the first palladium-catalyzed cross-coupling reaction of diazonaphthoquinone and arylboronic acid has been attempted, providing a novel access to biaryl compounds (Table 6).21 It was proved that, using Pd(OAc)2 as catalyst, instead of Pd(0), had a greater effect on the yield. In addition, using acetic acid as solvent made the reaction media more clean than using other solvent. Moreover, addition of fluoride anion was effective in such reaction as previously mentioned in Suzuki-Miyaura coupling reaction.22
The most believable reaction mechanism is shown in Scheme 6, which is the Pd(II)-catalytic cycle via the migratory insertion of a palladium carbene complex.23,24 The reaction is initiated by the transmetallation of arylboronic acid by the aid of F– and Pd(OAc)2 to generate intermediate I, which reacts with diazonaphthoquinone (2) to form palladium carbene complex II. Migratory insertion of the aryl group to the carbene carbon occurs, generating palladium complex III. Finally, protonation of acetic acid to III affords the coupling product (11) and regenerates Pd(OAc)2.
Diazonaphthoquinones act as aryl donor
Another application is that, the synthesis of the naphthofuran derivatives with its reported interesting biological activities.25 It was proved that dihydronaphthofuran derivatives could be synthesized by Rh-catalyzed intermolecular cycloaddition reaction of diazonaphthoquinones with enol ethers (12) (Scheme 7).26 No improvement of the yield was observed either by using Rh2(OCOCF3) or under Kraus reaction condition.27 Moreover, no cycloadduct product was formed in absence of Rh catalyst. In addition, it was observed that, 2-diazonaphthoquinone having an electron-donating group at C-4 position efficiently afforded dihydronaphthofuran in high yield.
Furthermore, this methodology was extended to the synthesis of α-arylcarbonyl compounds. The Rh2(OAc)4-catalyzed cycloaddition reaction of 2-diazonaphthoquiones with ketene acetals was examined and the obtained naphthofuran derivatives were then transformed to the corresponding α-naphthyl esters in high yield by treatment with tetrabutylammonium fluoride (TBAF) (Table 7). In entry 6, the formation of the corresponding lactone was observed with 6% yield.
It is worth noting to mention that a similar α-(1-hydroxy-2-naphthyl) ester was previously prepared from 1-naphthol in eight steps.28 Interestingly, this method provides more rapid synthesis via diazotization of 1-naphthol followed by Rh2(OAc)4-catalyzed reaction with ketene silyl acetal and finally ring opening with TBAF. To the best of our knowledge, this novel method was the first to describe that diazonaphthoquinones could be used as aryl donors for the metal-catalyzed α-arylation of ester enolate equivalents.
Pd-Catalyzed macrocyclization reaction with cyclic ether
Pd(OAc)2 was found to be an efficient catalyst for several coupling reactions of 1,2-diazonaphthoquinones. Unexpectedly, new method for the synthesis of medium-sized/macrocyclic ethers was developed, during the evaluation of the stability of 2-diazonaphthoquinone (2) when refluxed with catalytic amount of Pd(OAc)2 using various solvents (CH2Cl2, toluene, benzene, MeCN and THF).29 As a result, new method for the efficient synthesis of protected 1,2-naphthalenediols has been explored. As shown in Scheme 8, cyclic ethers (15) and (16) were obtained in reasonable yield using the determined optimum cyclization conditions. It was found that lower temperature (45 C) and using Rh, PdCl2 and Pd(OCOCF3)2 catalysts were not efficient for such cyclization. Moreover, no cyclic ethers were obtained upon the addition of a halide anion (LiCl) or during the use of Cu reagents, except for Cu(OTf)2 and Cu(OTf)·C6H5, a minor amount of C-H insertion product (17) was formed.
Next, during investigation of the Pd(OAc)2-catalyzed cyclization reaction using different diazonaphthoquinones substrates, it was found that introduction of a C-3 substituent was efficient for the selective formation of 8-membered ring cyclic ethers (15). On the other hand, the expected cyclization products were not generated using 4-methoxy-2-diazonaphthoquinone but only a dimerization product was obtained in 10% yield.
In addition, examination of Pd(OAc)2-catalyzed reaction of 2-diazonaphthoquinone with oxetane and tetrahydropyrane (THP) was proved to yield 15-membered cyclic ether (18) and a mixture of 9-membered cyclic ether (19) and 15-membered ether (20), respectively (Scheme 9). It was observed that the reactions of 1-diazonaphthoquinone proceeded smoothly in a similar manner.
Rh-Catalyzed intramolecular C-H insertion reaction
-Phenylnaphthalene lactones derivatives are widely used as intermediates for the synthesis of many bioactive natural products, such as polyketides and biaryl compounds (Figure 2).30
Although Pd-catalyzed intramolecular biaryl coupling of halogenated aryl carboxylic acid aryl esters was reported to be the most efficient method, it suffered from several problems such as a limited accessibility of starting haloaryl compounds, the reproducibility of the reaction and the harsh reaction conditions.31 We developed novel method for the synthesis of β-phenylnaphthalene lactones using Rh-catalyzed intramolecular formal C-H insertion cyclization reaction of 3-aryloxycarbonyldiazonaphthoquinones (Table 8).32
Judging from these results, it is apparent that there are two potential reaction sites for the formation of 6-membered ring in case of meta-monosubstituted phenyl esters. The C-C bond cyclization is favored at the para position of the substituent R due to less steric hindrance. In case of ortho-monosubstituted phenyl esters, regioselectivity of the present intramolecular Rh-catalyzed cyclization is largely dependent on the type of substituent on aromatic ring. Finally, the introduction of a methyl, phenyl or methoxy substituent at the 4-position to 3-aryloxycarbonyl-2-diazonaphthoquinone efficiently afforded the cyclized products in high yield.
Rh-Catalyzed reaction with indole
Baral et al. have developed a regioselective direct 3-arylation of indoles with 1-diazonaphthalen-2-(1H)-ones (1) by rhodium(II) pivalate-catalyzed cross-coupling reaction, yielding a variety of novel 3-naphthylindoles in high yield (eq. 5).33
Rh-Catalyzed reaction with benzyl/acyl halides
An efficient synthesis of different halonaphthalenyl ethers and esters was achieved via Rh(II)-catalyzed reaction of diazo compounds with benzyl halides or acid halides, respectively (eq.6)34
3-2. SYNTHETIC STUDY OF NATURAL COMPOUNDS
Synthetic study of kosinostatin
One of the most efficient applications of the recently developed diazo-transfer reaction is the synthesis of lactone (22), which corresponds to the BCDE ring fragment of kosinostatin aglycon (23).35,36 This aglycon (23) constitutes the core structure of the quinocycline/isoquinocyline antibiotics with their unique structure and several biological activities (Scheme 10).37
The D ring of lactone (22) was characterized with three carbon chiral centers, which assumed to be constructed by the oxidative cyclization of alkenyl carboxylic acid. Therefore, ester BCD ring (21) was set as its precursor and it was synthesized via two routes. First, compound (21) was synthesized from 2,5-dimethoxybenzaldehyde by the combination of typical known transformations including efficient application of non-aqueous OsO4 oxidation in the presence of PhB(OH)2.32 Unfortunately this pathway required 15 long steps and suffered from some difficulties. One of its main drawbacks was ortho-alkoxycarbonylmethylation of 1-naphthol. As a result, this process was improved by alkoxycarbonylmethylation using the recently developed diazonaphthoquinone. Scheme 11 illustrates that ester (21) was successfully synthesized in 9 steps from the same starting aldehyde applying Horner-Wittig reaction. By this new developed synthetic route, the number of reaction steps was reduced to 9 steps and the total yield was increased to 8.6% instead of 3.4%. Finally, 21 was stereoselectively converted to lactone (22) via trifluoroacetic acid-mediated cyclization of the 3,4-epoxy- cyclohexanecarboxylic acid derivative.
Synthetic study of pradimicinone
Another interesting application was the synthesis of pradimicinone (25).32 As shown in Scheme 12, the diazo-transfer reaction of ADMC (3) to polysubstituted 1-naphthol afforded diazonaphthoquinone in the presence of 15-crown-5. Then Rh-catalyzed cyclization reaction followed by reduction of the formed lactone afforded triol, successfully. According to previously reported literature, this triol underwent several transformations to yield pradimicinone (25).
4. CONCLUSION
Here, we briefly reviewed the current progress of the synthetic chemistry on diazonapthoquiones, which are efficiently prepared through short direct pathway and have high chemical reactivity toward several types of metal-catalyzed reactions, such as O-H or C-H insertion reaction. Further studies on more wide applications of diazo-transfer reactions are in progress. This survey attempts to summarize the synthetic methods, reactions and applications for future studies to be done in the same field.
ACKNOWLEDGEMENTS
We thank Prof. Tatsuo Okauchi (Kyushu Institute of Technology) for his helpful discussion.
References
01. V. V. Ershov, G. A. Nikiforov, and C. R. H. I. de Jonge, Quinone Diazides, Elsevier, Amsterdam, 1981.
2. a) M. E. Bodini and V. Arancibia, Trans. Met. Chem., 1997, 22, 150; CrossRef b) M. C. Foti, E. R. Johnson, M. R. Vinqvist, J. S. Wright, L. R. C. Barclay, and K. U. Ingold, J. Org. Chem., 2002, 67, 5190; CrossRef c) E. Pino, A. Aspée, C. López-Alarcón, and E. Lissi, J. Phys. Org. Chem., 2006, 19, 867. CrossRef
3. a) C. R. Rice, M. D. Ward, M. K. Nazeeruddin, and M. Grätzel, New J. Chem., 2000, 24, 651; CrossRef b) R. Mosurkal, J.-A. He, K. Yang, L. A. Samuelson, and J. Kumar, J. Photochem. Photobiol. A: Chem., 2004, 168, 191; CrossRef c) T. Lu, P. Shao, I. Mathew, A. Sand, and W. Sun, J. Am. Chem. Soc., 2008, 130, 15782; CrossRef d) I. Hod, M. Shalom, Z. Tachan, S. Rühle, and A. Zaban, J. Phys. Chem. C, 2010, 114, 10015; CrossRef e) B.-K. An, W. Hu, P. L.Burn, and P. Meredith, J. Phys. Chem. C, 2010, 114, 17964; CrossRef f) R. Sánchez-de-Armas, J. Oviedo, M. Á. S. Miguel, and J. F. Sanz, J. Phys. Chem. C, 2011, 115, 11293. CrossRef
4. a) A. Reiser, H. Y. Shih, T. F. Yeh, and J. P. Huang, Angew. Chem., 1996, 108, 2610; CrossRef A. Reiser, H. Y. Shih, T. F. Yeh, and J. P. Huang, Angew. Chem. Int. Ed. Engl. 1996, 35, 2428; CrossRef b) A. Reiser, J. P. Huang, X. He, T. F. Yeh, S. Jha, H. Y. Shih, M. S. Kim, Y. K. Han, and K. Yan, Eur. Polym. J., 2002, 38, 619; CrossRef c) K. i. Fukukawa and M. Ueda, Polym. J., 2008, 40, 281. CrossRef
5. a) M. Yagihara, Y. Kitahara, and T. Asao, Chem. Lett., 1974, 3, 1015; CrossRef b) N. P. Hacker and N. J. Turro, Tetrahedron Lett., 1982, 23, 1771; CrossRef c) G. Bucher and W. Sander, J. Org. Chem., 1992, 57, 1346; CrossRef d) J. I. K. Almstead, B. Urwyler, and J. Wirz, J. Am. Chem. Soc., 1994, 116, 954; CrossRef e) G. G. Qiao, J. Andraos, and C. Wentrup, J. Am. Chem. Soc., 1996, 118, 5634; CrossRef f) N. C. de Lucas, J. C. Netto-Ferreira, J. Andraos, J. Lusztyk, B. D. Wagner, and J. C. Scaiano, Tetrahedron Lett., 1997, 38, 5147; CrossRef g) S. Murata, J. Kobayashi, C. Kongou, M. Miyata, T. Matsushita, and H. Tomioka, J. Org. Chem., 2000, 65, 6082; CrossRef h) W. Kirmse, Eur. J. Org. Chem., 2002, 14, 2193; CrossRef i) N. K. Urdabayev and V. V. Popik, J. Am. Chem. Soc., 2004, 126, 4058. CrossRef
6. K. Bahadur, Somia Magar, and Y. R. Lee, Org. Lett., 2013, 15, 4288.
7. a) L. C. Anderson and M. J. Roedel, J. Am. Chem. Soc., 1945, 67, 955; CrossRef b) J. D. C. Anderson, R. J. W. Le Fevre, and I. R. Wilson, J. Chem. Soc., 1949, 2082; CrossRef c) W. Sander, G. Bucher, H. Wandel, E. Kraka, D. Cremer, and W. S. Sheldrick, J. Am. Chem. Soc., 1997, 119, 10660. CrossRef
8. a) M. P. Cava, R. L. Litle, and D. R. Napier, J. Am. Chem. Soc., 1958, 80, 2257; CrossRef b) N. P. Hacker and N. J. Turro, Tetrahedrone Lett. 1982, 23, 1771; CrossRef c) P. J. N. Brown, J. I. G. Cadogan, I. Gosney, A. Johnstone, R. M. Paton, and N. H. Wilson, J. Chem. Soc., Perkin Trans. 2, 1996, 2303; CrossRef d) V. F. Ferreira, A. Jorqueira, K. Z. Leal, H. R. X. Pimentel, P. R. Seidl, M. N. da Silva, M. C. B. V. da Silva, M. C. B. V. da Souza, A. V. Pinto, J. L.Wardell, and S. M. S. V. Wardell, Magn. Reson. Chem., 2006, 44, 481. CrossRef
9. a) T. Ye and M. A. Mckervey, Chem. Rev., 1994, 94, 1091; CrossRef b) Z. Zhang and J. Wang, Tetrahedron, 2008, 64, 6577; CrossRef c) M. Regitz, Angew. Chem., Int. Ed. Engl., 1967, 6, 733; CrossRef d) A. Padwa and D. J. Austin, Angew. Chem., Int. Ed. Engl., 1994, 33, 1797. CrossRef
10. H. Balli, V. Muller, and A. Sezen-Gezgin, Helv. Chim. Acta, 1978, 61, 104. CrossRef
11. M. Kitamura, J. Synth. Org. Chem. Jpn., 2014, 72, 14. CrossRef
12. Diazo-transfer to 1,3-carbonyl compounds, see: a) M. Kitamura, N. Tashiro, and T. Okauchi, Synlett, 2009, 2943; CrossRef b) M. Kitamura, N. Tashiro, S. Miyagawa, and T. Okauchi, Synthesis, 2011, 1037. CrossRef
13. a) M. Kitamura, M. Yano, N. Tashiro, S. Miyagawa, M. Sando and T. Okauchi, Eur. J. Org. Chem., 2011, 3, 458; CrossRef b) M. Kitamura, S. Kato, M. Yano, N. Tashiro, Y. Shiratake, M. Sando, and T. Okauchi, Org. Biomol. Chem., 2014, 12, 4397. CrossRef
14. a) M. Kitamura, N. Tashiro, R. Sakata, and T. Okauchi, Synlett, 2010, 2503; CrossRef b) M. Kitamura, R. Sakata, N. Tashiro, A. Ikegami, and T. Okauchi, Bull. Chem. Soc. Jpn., 2015, 88, 824. CrossRef
15. a) M. Kitamura, N. Tashiro, Y. Takamoto, and T. Okauchi, Chem. Lett., 2010, 39, 732; CrossRef b) M. Kitamura, T. Koga, M. Yano, and T. Okauchi, Synlett, 2012, 1335; CrossRef c) M. Kitamura, K. Murakami, Y. Shiratake, and T. Okauchi, Chem. Lett., 2013, 42, 691. CrossRef
16. Unpublished results.
17. a) P. Stahl, L. Kissau, R. Mazitschek, A. Huwe, P. Furet, A. Giannis, and H. Waldmann, J. Am. Chem. Soc., 2001, 123, 11586; CrossRef b) T. Lu, P. Shao, I. Mathew, A. Sand, and W. Sun, J. Am. Chem. Soc., 2008, 130, 15782; CrossRef c) S. Madan and C. H. Cheng, J. Org. Chem., 2006, 71, 8312. CrossRef
18. a) K. L. Platt and F. Oesch, J. Org. Chem., 1983, 48, 265; CrossRef b) J. L. Zambrano and R. Dorta, Synlett, 2003, 1545; CrossRef c) J. K. Crandall, M. Zucco, R. S. Kirsch, and D. M. Coppert, Tetrahedron Lett., 1991, 32, 5441. CrossRef
19. M. Kitamura, M. Kisanuki, R. Sakata, and T. Okauchi, Chem. Lett., 2011, 40, 1129. CrossRef
20. M. Kitamura, M. Kisanuki, and T. Okauchi, Eur. J. Org. Chem., 2012, 5, 905. CrossRef
21. M. Kitamura, R. Sakata, and T. Okauchi, Tetrahedron Lett., 2011, 52, 1931. CrossRef
22. a) S. W. Wright, D. L. Hageman, and L. D. McClure, J. Org. Chem., 1994, 59, 6095; CrossRef b) T. Kirschbaum, C. A. Briehn, and P. Bäuerle, J. Chem. Soc., Perkin Trans. 1, 2000, 1211. CrossRef
23. C. Peng, Y. Wang, and J. Wang, J. Am. Chem. Soc., 2008, 130, 1566. CrossRef
24. a) K. L. Greenman, D. S. Carter, and D. L. Van Vranken, Tetrahedron, 2001, 57, 5219; CrossRef b) K. L. Greenman and D. L. Van Vranken, Tetrahedron, 2005, 61, 6438; CrossRef c) S. K. J. Devine and D. L.Van Vranken, Org. Lett., 2007, 9, 2407; CrossRef d) J. Barluenga, P. Moriel, C. Valdés, and F. Aznar, Angew. Chem. Int. Ed., 2007, 46, 5587; CrossRef e) S. Chen and J. Wang, Chem. Commun., 2008, 4198; CrossRef f) Q. Xiao, J. Ma, Y. Yang, Y. Zhang, and J. Wang, Org. Lett., 2009, 11, 4732; CrossRef g) Z. Zhang, Y. Liu, M. Gong, X. Zhao, Y. Zhang, and J. Wang, Angew. Chem. Int. Ed., 2010, 49, 1139; CrossRef h) A. Khanna, I. D. U. A. Premachandra, P. D. Sung, and D. L. Van Vranken, Org. Lett., 2013, 15, 3158; CrossRef i) A. Khanna, I. D. U. A. Premachandra, P. D. Sung, and D. L. Van Vranken, Org. Lett., 2013, 15, 3694; CrossRef j) E. S. Gutman, V. Arredondo, and D. L. Van Vranken, Org. Lett., 2014, 16, 5498; CrossRef k) M. Kitamura, R. Yuasa, and D. L. Van Vranken, Tetrahedron Lett., 2015, 56, 3027; CrossRef l) I. D. U. A. Premachandra, T. Nguyen, C. Shen, E. Gutman, and D. L. Van Vranken, Org. Lett., 2015, 17, 5464. CrossRef
25. V. S. P. R. Lingam, D. H. Dahale, K. Mukkanti, B. Gopalan, and A. Thomas, Tetrahedron Lett., 2012, 53, 5695. CrossRef
26. M. Kitamura, K. Araki, H. Matsuzaki, and T. Okauchi, Eur. J. Org. Chem., 2013, 23, 5045. CrossRef
27. G. A. Kraus, J. O. Nagy, and J. DeLano, Tetrahedron, 1985, 41, 2337. CrossRef
28. M. Yoshida, M. Higuchi, and K. Shishido, Org. Lett., 2009, 11, 4752. CrossRef
29. M. Kitamura, M. Kisanuki, K. Kanemura, and T. Okauchi, Org. Lett., 2014, 16, 1554. CrossRef
30. a) N. Ueberschaar, Z. Xu, K. Scherlach, M. Metsa-Ketela, T. Bretschneider, H.-M. Dahse, H. Görls, and C. Hertweck, J. Am. Chem. Soc., 2013, 135, 17408; CrossRef b) S. Horii, H. Fukase, E. Mizuta, K. Hatano, and K. Mizuno, Chem. Pharm. Bull., 1980, 28, 3601; CrossRef c) G. Bringmann and D. Menche, Angew. Chem. Int. Ed., 2001, 40, 1687; CrossRef d) T. Matsumoto, T. Hosoya, and K. Suzuki, J. Am. Chem. Soc., 1992, 114, 3568; CrossRef e) A. Hager, D. Mazunin, P. Mayer, and D. Trauner, Org. Lett., 2011, 13, 1386; CrossRef f) M. Tamiya, K. Ohmori, M. Kitamura, H. Kato, T. Arai, M. Oorui, and K. Suzuki, Chem. Eur. J., 2007, 13, 9791; CrossRef g) M. Kitamura, K. Ohmori, T. Kawase, and K. Suzuki, Angew. Chem. Int. Ed., 1999, 38, 1229; CrossRef h) K. Ohmori, M. Tamiya, M. Kitamura, H. Kato, M. Oorui, and K. Suzuki, Angew. Chem. Int. Ed., 2005, 44, 3871. CrossRef
31. a) T. Harayama and H. Yasuda, Heterocycles, 1997, 46, 61; CrossRef b) Q. J. Zhou, K. Worm, and R. E. Dolle, J. Org. Chem., 2004, 69, 5147; CrossRef c) H. Abe, K. Nishioka, S. Takeda, M. Arai, Y. Takeuchi, and T. Harayama, Tetrahedron Lett., 2005, 46, 3197; CrossRef d) S. R. Taylor, A. T. Ung, and S. G. Pyne, Tetrahedron, 2007, 63, 10889; CrossRef e) O. C. Arellano and A. C. Vargas, Tetrahedron Lett., 2010, 51, 602; CrossRef f) J. Luo, Y. Lu, S. Liu, J. Liu, and G. Denga, Adv. Synth. Catal., 2011, 353, 2604; CrossRef g) S. Luo, F. X. Luo, X. S. Zhang, and Z. J. Shi, Angew. Chem. Int. Ed., 2013, 52, 10598. CrossRef
32. M. Kitamura, S. Takahashi, and T. Okauchi, J. Org. Chem., 2015, 80, 8406. CrossRef
33. E. R. Baral, Y. R. Lee, and S. H. Kim, Adv. Synth. Catal., 2015, 357, 2883. CrossRef
34. E. R. Baral, Y. R. Lee, S. H. Kim, and Y. J. Wee, Synthesis, 2016, 48, 579. CrossRef
35. M. Kitamura, K. Kubo, S. Yoshinaga, H. Matsuzaki, K. Ezaki, T. Matsuura, D. Matsuura, N. Fukuzumi, K. Araki, and M. Narasaki, Tetrahedron Lett., 2014, 55, 1653. CrossRef
36. a) J. Cordes, K. Harms, and U. Koert, Org. Lett., 2010, 12, 3808; CrossRef b) M. A. Breuning, K. Harms, and U. Koert, Org. Lett., 2011, 13, 1402; CrossRef c) M. Dischmann, T. Frassetto, M. A. Breuning, and U. Koert, Chem. Eur. J., 2014, 20, 11300. CrossRef
37. a) D. B. Cosulich, J. H. Mowat, R. W. Broschard, J. B. Patrick, W. E. Meyer, Tetrahedron Lett., 1964, 3, 453; CrossRef b) J. H. Mowat, R. W. Broschard, J. B. Patrick, W. E. Meyer, Tetrahedron Lett., 1964, 5, 750; CrossRef c) A. Tulinsky, J. Am. Chem. Soc., 1964, 86, 5368; CrossRef d) T. Furumai, Y. Igarashi, H. Higuchi, N. Saito, and T. Oki, J. Antibiot., 2002, 55, 128; CrossRef e) Y. Igarashi, H. Higuchi, T. Oki, and T. Furumai, J. Antibiot., 2002, 55, 134; CrossRef f) M. Y. El-Naggar, J. Microbiol., 2007, 45, 262