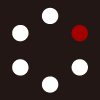
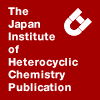
HETEROCYCLES
An International Journal for Reviews and Communications in Heterocyclic ChemistryWeb Edition ISSN: 1881-0942
Published online by The Japan Institute of Heterocyclic Chemistry
e-Journal
Full Text HTML
Received, 9th November, 2015, Accepted, 4th December, 2015, Published online, 17th December, 2015.
DOI: 10.3987/REV-15-829
■ Applications of C-H Insertion Reaction in Total Synthesis of Biologically Active Heterocyclic Natural Products
Toshiyuki Kan,* Tomohiro Asakawa, and Makoto Inai
Synthetic Organic & Medicinal Chemistry, School of Pharmaceutical Science, University of Shizuoka, 52-1 Yada, Suruga, Shizuoka 422-8526, Japan
Abstract
Here, we summarize our work on applications of the Rh-catalyzed C-H insertion reaction to syntheses of heterocyclic natural products. Total synthesis of racemic serotobenine (4) was achieved in an optical active form. Enantioselective synthesis of SB-203207 (25) was accomplished via desymmetric C-H insertion reaction of 33 to construct the bicyclo[3.3.0] framework. Stereoselective synthesis of MFPA (53) was accomplished with the aid of an intermolecular C-H insertion reaction. Synthesis of aperidine (70) featured cis-selective construction of the dihydrobenzofuran ring of 81 by utilizing Hashimoto’s catalyst.INTRODUCTION
During the course of our development of the Ns-strategy1,2 for the construction of medium-size rings, we completed a total synthesis of ephedradine A (1).3 During the synthesis, we found that Rh-carbenoid-mediated C-H insertion reaction4 enabled efficient construction of the dihydrobenzofuran ring. As shown in Scheme 1, treatment of diazoester 2 bearing a chiral auxiliary with Rh2(S-DOSP)4 catalyst5 resulted in efficient C-H insertion to provide the desired dihydrobenzofuran 3.6 This transformation can easily be scaled up to the level of hundreds of grams. Furthermore, there was no requirement for an electrophilic functional group for the carbon-carbon bond formation. Thus, the introduction of our C-H insertion reaction considerably streamlined the synthetic strategy. Inspired by this finding, we subsequently employed the C-H insertion reaction in the synthesis of several other complex natural products.
RESULTS AND DISCUSSION
1. Total synthesis of serotobenine (4)
Serotobenine (4) was isolated from safflower seeds as a racemic natural product in 1985.7 Recently, another related natural product, decursivine (5),8 was isolated in optically active form from Rhaphidophora decursiva. Because the biosynthetic pathways of 4 and 5 should be similar, a special mechanism may operate for racemization of 4. We hypothesized that serotobenine (4) might racemize through the quinonemethide intermediate 6, and aimed to synthesize optically active 4 and 5 to solve the mystery.
Serotobenine (4) and decursivine (5) are composed of an indole, an eight-membered lactam, and a dihydrobenzofuran ring, and the order of construction of the heterocyclic ring is likely to be important. As shown in Scheme 2, the synthesis was started with construction of the indole skeleton. After O-allylation of 3-methyl-4-nitrophenol (7), the Leimgruber-Batcho procedure9 provided 5-allyloxy-1H-indole (8). After protection of 8 with a Ts group, thermal Claisen rearrangement provided 9 in a regio-selective manner.10 Benzyl halide derivative 10 was incorporated into the resultant phenol, and then oxidative cleavage of the olefin and NaClO2-mediated oxidation furnished carboxylic acid 11. After incorporation of a chiral auxiliary (Xm) into 11, ABSA-mediated diazotransfer reaction afforded 12. The key C-H insertion reaction was accomplished by treatment of diazoester 12 with Rh2(S-DOSP)4 catalyst to provide dihydrobenzofuran ring 13. In this reaction, we found that mandelate-amide (Xm*) was superior to lactate-amide as a chiral auxiliary.
Next, incorporation of a side chain at the 3-position of indole 13 was achieved by bromination, and Pd-mediated Stille-type coupling reaction provided the desired 14. The allyl group was converted to ethyl azide 15 via a five-step sequence. Removal of the chiral auxiliary and condensation of the resultant carboxylic acid 16 with pentafluorophenol gave activated ester 17. Upon treatment of azide 17 with PPh3 in the presence of H2O, reduction to the amine and simultaneous cyclization proceeded to afford eight-membered lactam 18. Removal of the Ts group and cleavage of the benzyl ether under hydrogenolysis conditions yielded (–)-serotobenine (4).11
Synthesis of decursivine (5) was also accomplished, as shown in Scheme 4. Construction of the dihydrobenzofuran ring of 19 again utilized the C-H insertion reaction. After hydrolysis of the chiral auxiliary and Ts group of 19, condensation with Pfp-OH provided the activated ester 20. Treatment of indole 20 and nitro-olefin 21 in the presence of methanesulfonic acid, followed by Michael reaction, and elimination of dimethylamine proceeded smoothly to give 22. Subsequent treatment of 22 under hydrogenolysis conditions, reduction of both the nitro functionality and double bond, and subsequent amide bond formation proceeded to provide decursivine (5) in a single step.
With optically active 4 and 5 in hand, we examined their optical stability under various conditions. Neither racemization nor epimerization occurred upon exposure of 1 to acidic or basic conditions. On the other hand, on treatment of N-Ts derivative 23 with Cs2CO3, a remarkable decrease of the enantiomeric excess was observed. Since incorporation of the Ts group increased the leaving ability of the 5-hydroxyindole group, the ring-opening reaction of dihydrobenzofuran would readily occur to afford p-quinonemethide intermediate 6. Furthermore, the acidic α-proton of the amide should equilibrate. On the other hand, the methylenedioxy bridge likely prevents conversion of 24 to the p-quinonemethide intermediate, which may be the reason why 5 is optically active, whereas natural 4 exists as a racemic mixture.11
2. Total synthesis of SB-203207 (25)
SB-203207 (25) was isolated from a Streptomyces species by a SmithKline Beecham group. It strongly inhibits isoleucyl tRNA synthetase.12 A unique structural feature of 25 is the -hydroxyl -disubstituted--amino acid moiety on the 6-aza indene skeleton. In 1995, Kende and co-workers reported a total synthesis of altemicidin, the core compound of 25,13 but 25 itself has not been synthesized.
The heart of our synthetic plan for 25 is illustrated in Scheme 6. The highly polar sulfonamide and vinylogous urea of 25 would be introduced at a later stage of the synthesis. Because incorporation of nitrogen onto the quaternary carbon would be performed by Curtius rearrangement, the azabicyclo[4.3.0]skeleton 26 would serve as a key intermediate. The cyclic enamide of 26 would be constructed from cyclopentene 27 by oxidative cleavage of the double bond followed by regioselective introduction of nitrogen and re-cyclization. The quaternary carbon and the secondary alcohol would be stereoselectively installed by employing -ketoester 28. Synthesis of the optically active bicyclo[3.3.0] framework 28 would be accomplished by using our Rh-carbenoid mediated intramolecular C-H insertion reaction14 of 29.
Since direct incorporation of a chiral auxiliary (Xm*-OH) into β-ketoester 33 was difficult, we started the synthesis from 30, as shown in Scheme 7. According to Fukuyama’s procedure,15 preparation of the diazo group was performed by treatment of α-bromoester 30 and bistosyl hydrazine with DBU to give 31. Then, condensation of 31 and aldehyde 3214 by means of a modified Roskamp reaction16 provided the C-H insertion precursor 33. Treatment of 33 with 0.1 mol% Rh2(R-DOSP)4 resulted in desymmetric C-H insertion to afford the bicyclo[3.3.0]octane ring compound 34. This transformation had the advantage of enabling one-step generation of the two chiral centers at the ring fusion. After conversion to the allyl ester 35, stereoselective hydroxymethylation was achieved by treatment with formalin and a catalytic amount of KHCO3 to give 36. Subsequent stereoselective reduction of 36 with NaBH(OAc)317 gave 37. As expected, alkylation occurred from the convex face of 35 and reduction proceeded via chelation with the primary hydroxyl group of 36.
As shown in Scheme 8, the primary alcohol and secondary alcohol were protected as TBDPS and MOM ether, respectively. Because directly installing the nitrogen group is difficult, oxidative cleavage of the double bond of 38 and intermolecular delivery of nitrogen were employed. Deprotection of the allyl group of 38,18 conversion to the acid chloride, and treatment with ammonia gave amide 39. After dihydroxylation of 39, treatment with Pb(OAc)4 generated dialdehydes, where the amide nitrogen selectively attacked the closer aldehyde to provide hemiaminal 40. After conversion to the dimethyl acetal and methyl hemiaminal, selective reduction of hemiaminal 41 was achieved by treatment with NaBH3CN in the presence of formic acid to give lactam 42. Opening of the gamma lactam of 42 was achieved by combining activation by Ns imide and participation of the neighboring primary alcohol through the lactam intermediate to provide 43. Cyclic enamide 44 was obtained by heating 43 with silica gel.
Next, introduction of a nitrogen atom onto the quaternary carbon of 44 was performed by treatment with DPPA19 to give 45, as shown in Scheme 9. After incorporation of a C1 unit into enamide 45 via modified Vilsmeier reaction20 and protection of 46 with a Boc group, deprotection of the Ns group and N-methylation afforded 47. Conversion of aldehyde 47 to nitrile 48 was performed by treatment with hydroxylamine, followed by addition of acetic anhydride. Although conversion to aldehyde 49 proceeded smoothly via hydrolysis of the oxazolidinone ring and Dess-Martin periodinane (DMP) oxidation, further oxidation to the corresponding acid derivative was difficult.
Since typical NaOCl2-mediated Kraus oxidation, as well as TEMPO- or AZADO-catalyzed oxidation, resulted in decomposition of the reactive cyanoenamide group, oxidation of 49 required non-nucleophilic reaction conditions. We found that oxidation of the cyanohydrin intermediate was suitable. After conversion to cyanohydrin by treatment with Et2AlCN,21 DMP-mediated oxidation provided 50 without any decomposition of the enamide group. After hydrolysis of acylcyanide 50, treatment with diphenyl- diazomethane provided 51. Simultaneous removal of the Boc and MOM groups of 51 was achieved by treatment with B-bromocatechol borane and incorporation of the side chain gave 52. Conversion of the cyanoenamide 52 to carbamoylenamide was performed in the presence of Parkins catalyst.22 Finally, simultaneous cleavage of the Cbz and diphenylmethyl ester groups under hydrogenolysis conditions yielded SB-203207 (25).
3. Total synthesis of methoxyphenylkainic acid (MFPA: 53)
Kainoids have received significant attention due to their binding affinity for ionotropic glutamate receptors (iGluRs), which are involved in important neurophysiological processes, including memory and learning.23 Although many synthetic investigations of kainoids have been reported to date, efficient synthetic methods are still urgently required. During pioneering investigations of the potent natural product acromelic acid A (53), discovered by the Shirahama group,24 it was discovered that a synthetic MFPA (54) possessed more potent activity than 53.25 Inspired by this interesting structure–activity relationship information, we launched an investigation with the aim of developing efficient synthetic methods for 53.
Initially, we examined intramolecular C-H insertion reaction of diazoester 55, which was derived from condensation of phenylacetic acid and glutamic acid. However, the desired C-H insertion reaction did not proceed on treatment of 55 with Rh catalyst, and a complex mixture was formed. On the other hand, intermolecular C-H insertion reaction of the similar aryl diazoester 56 with cyclohexadiene proceeded smoothly to give 57, though the enantioselectivity was not satisfactory.26 In order to improve the selectivity, we prepared diazoester 58 bearing our chiral auxiliary. Upon treatment of 58 and cyclohexadiene with Rh2(R-DOSP)4 catalyst, the C-H insertion reaction proceeded smoothly to afford 59 exclusively. After conversion of 59 to the corresponding methyl ester, mono-selective oxidative cleavage of the diene was accomplished by ozonolysis at –78 °C. After confirmation of disappearance of the starting material, addition of NaBH4 to the ozonide intermediate 60 gave the corresponding primary alcohol 61 as a 1:1 mixture. This was converted to the lactone 62, and oxidative cleavage of the olefin proceeded with concomitant epimerization at the α-position of the aldehyde to give 63 predominantly. In this reaction, the absence of triethylamine base resulted in a 1:1 mixture. Without purification, aldehyde 63 was protected with ethylene glycol to give 64.
Reduction of the lactone 64 with DIBAL and conversion to the acetate gave 65. Upon treatment of 65 with Ns-NH227 in the presence of BF3·OEt2, amination reaction proceeded smoothly to afford 66. Selective reduction of the aminal of 66 was performed by treatment with DIBAL to give 67. Treatment of 67 under acidic conditions, alcoholysis of the acetal, and subsequent cyclization with the Ns-amide smoothly afforded the aminal as a single isomer. On treatment of the aminal with BF3·OEt2 and TMSCN, Strecker-type reaction occurred from the less-hindered b-face of the pyrrolidine ring to provide the aminonitrile 68 as a single diastereomer. Next, another nitrile group was incorporated by Mitsunobu reaction of acetone cyanohydrin and DMEAD,28 to afford 69. After deprotection of the Ns group by treatment with PhSH and base, acidic hydrolysis of the cyano groups was carried out in a sealed tube to give the desired MFPA (54).29
4. Total synthesis of aperidine (70)
From ancient times, beer has been a familiar drink with well-known gastrointestinal prokinetic effects. Professor Wakimoto, a former group-member and colleague of ours, isolated aperidine (70) and hordatine (71)30 from beer as compounds with muscarinic M3 receptor-binding activity.31 These compounds also exhibited α1 adrenoceptor antagonist activity. Although a total synthesis of hordatine (71) has been reported by Wakimoto et al.,32 they found difficulty in synthesizing 70, which was unstable due to its ready isomerization to the trans isomer 71.
Initially, we attempted to incorporate the side chain after construction of the dihydrobenzofuran ring by C-H insertion reaction, as shown in Scheme 13. Treatment of dihydrobenzofuran 72 and amine (R-NH2) with Me3Al resulted in epimerization at the C-2 position prior to the desired amide bond formation to give 73. This reaction presumably proceeded through Lewis acid-mediated p-quinonemethide intermediate formation. Next, hydrolysis of 72 by treatment with LiOH proceeded, with concomitant epimerization at the a-position of the ester group, to give 74. Therefore, we concluded that the labile cis-dihydrobenzofuran ring should be constructed in a late stage of the total synthesis.
As shown in Scheme 14, the cis-dihydrobenzofuran ring was constructed after incorporation of side chains. After Heck reaction of methyl ester 75 and methyl acrylate and hydrolysis of the diester, condensation of the resultant carboxylic acid 76 with butanol amine gave the amide 77. ABSA-mediated diazo transfer reaction of 77 did not proceed smoothly due to the lower pKa of the a-position of the amide group. Recently, Raines and coworkers reported an efficient conversion of an azide functionality to a diazo group using a novel phosphine reagent 80.33
Incorporation of an azide group was performed by introduction of a bromine atom into 77 and displacement of bromide with NaN3. Upon treatment of 79 with 80, phosphine-mediated activation of the azide group and subsequent diazoamide formation proceeded to provide 81. Construction of the cis-dihydrobenzofuran 82 was accomplished by treatment of 81 with Hashimoto catalyst Rh2(S-PTTL)4,34 to provide 82 in 75% yield. After deprotection of both TBS ethers of 82 by NH4F, a guanidine moiety was incorporated by means of the Mitsunobu reaction. Upon treatment of the di-Boc guanidine (83) in the presence of the corresponding alcohol with DEAD and PPh3, smooth amination proceeded to provide the protected aperidine 84. Finally, simultaneous removal of the Bn ether and Boc groups was performed by treatment with BCl3 at –78 °C. Careful work-up afforded 70.35
In this review, we have summarized our work on applications of the Rh-carbenoid-mediated C-H insertion reaction as a key step in total syntheses of several biologically active natural products. These synthetic studies have not only contributed to our understanding of the biological activities of the natural products, but also have uncovered the factors underlying other chemical behaviors of the compounds.
References
1. Review for Ns–strategy: (a) T. Kan and T. Fukuyama, J. Synth. Org. Chem. Jpn., 2001, 59, 779; CrossRef (b) T. Kan and T. Fukuyama, Chem. Commun., 2004, 35.
2. (a) A. Fujiwara, T. Kan, and T. Fukuyama, Synlett, 2000, 1667; CrossRef (b) T. Kan, H. Kobayashi, and T. Fukuyama, Synlett, 2002, 697. CrossRef
3. (a) W. Kurosawa, T. Kan, and T. Fukuyama, J. Am. Chem. Soc., 2003, 125, 8112; CrossRef (b) W. Kurosawa, H. Kobayashi, T. Kan, and T. Fukuyama, Tetrahedron, 2004, 60, 9615. CrossRef
4. For a review on C-H insertion reactions: (a) M. P. Doyle, M. A. Mckervey, and M. T. Ye, Modern Catalytic Methods for Organic Synthesis with Diazo Compounds: From Cyclopropanes to Ylides, Willey, New York, 1998; (b) H. M. L. Davies and R. E. J. Beckwith, Chem. Rev., 2003, 103, 2861. CrossRef
5. (a) H. M. L. Davies and T. Hansen, J. Am. Chem. Soc., 1997, 119, 9075; CrossRef (b) H. M. L. Davies and E. G. J. Antoulinakis, Organomet. Chem., 2001, 47, 617; (c) H. M. L. Davies and E. G. Antoulinakis, Org. Lett., 2000, 2, 4153; CrossRef (d) H. M. L. Davies, T. Hansen, and M. R. Churchill, J. Am. Chem. Soc., 2000, 122, 3063. CrossRef
6. W. Kurosawa, T. Kan, and T. Fukuyama, Synlett, 2003, 1028. CrossRef
7. H. Sato, H. Kawagishi, T. Nishimura, S. Yoneyama, Y. Yoshimoto, S. Sakamura, A. Furusaki, S. Katsuragi, and T. Matsumoto, Agric. Biol. Chem., 1985, 49, 2969. CrossRef
8. H. Zhang, S. Qiu, P. Tamez, G. T. Tan, Z. Aydogmus, N. Van Hung, N. M. Cuong, C. Angerhofer, D. D. Soejarto, J. M. Pezzuto, and H. H. S. Fong, Pharm. Biol., 2002, 40, 221. CrossRef
9. A. D. Batcho and W. Leimgruber, Org. Synth. Coll. Vol. VII, 1990, 34.
10. C. J. Moody, J. Chem. Soc., Perkin Trans. 1, 1984, 1333. CrossRef
11. Y. Koizumi, H. Kobayashi, T. Wakimoto, T. Furuta, T. Fukuyama, and T. Kan, J. Am. Chem. Soc., 2008, 130, 16854. CrossRef
12. (a) M. G. Banwell, C. F. Crasto, C. J. Easton, A. K. Forrest, T. Karoli, D. R. March, L. Mensah, M. R. Nairn, P. J. O’Hanlon, M. D. Oldham, and W. Yue, Bioorg. Med. Chem. Lett., 2000, 10, 2263; CrossRef (b) M. G. Banwell, C. F. Crasto, C. J. Easton, T. Karoli, D. R. March, M. R. Nairn, P. J. O’Hanlon, M. D. Oldham, A. C. Willis, and W. Yue, Chem. Commun., 2001, 2210; CrossRef (c) C. F. Crasto, A. K. Forrest, T. Karoli, D. R. March, L. Mensah, P. J. O’Hanlon, M. R. Nairn, M. D. Oldham, W. Yue, M. G. Banwell, and C. J. Easton, Bioorg. Med. Chem., 2003, 11, 2687. CrossRef
13. A. S. Kende, K. Liu, and K. M. Jos Brands, J. Am. Chem. Soc., 1995, 117, 10597. CrossRef
14. T. Kan, Y. Kawamoto, T. Asakawa, T. Furuta, and T. Fukuyama, Org. Lett., 2008, 10, 169. CrossRef
15. T. Toma, J. Shimokawa, and T. Fukuyama, Org. Lett., 2007, 9, 3195. CrossRef
16. (a) S. R. Angle, D. Bensa, and D. S. Belanger, J. Org. Chem., 2007, 72, 5592; CrossRef (b) C. R. Holmquist and E. J. Roskamp, J. Org. Chem., 1989, 54, 3258. CrossRef
17. D. Seebach, E. Hüngerbuhler, P. Schnurrenberger, B. Weidmann, and M. Züger, Synthesis, 1982, 138. CrossRef
18. D. A. Evans, K. T. Chapman, and E. M. Carreira, J. Am. Chem. Soc., 1988, 110, 3560. CrossRef
19. T. Shioiri, K. Ninomiya, and S. Yamada, J. Am. Chem. Soc., 1972, 94, 6203. CrossRef
20. W. Nagata, M. Yoshioka, and S. Hirai, Tetrahedron Lett., 1962, 3, 461. CrossRef
21. L. I. Smith and K. L. Howard, Org. Synth, Coll. Vol. III, 1995, 351.
22. T. Ghaffar and A. W. Parkins, J. Mol. Catal. A, 2000, 160, 249. CrossRef
23. Reviews for kainoids: (a) M. G. Moloney, Nat. Prod. Rep., 2002, 19, 597; CrossRef (b) M. G. Moloney, Nat. Prod. Rep., 1999, 16, 485; CrossRef (c) M. G. Moloney, Nat. Prod. Rep., 1998, 15, 205; CrossRef (d) A. F. Parsons, Tetrahedron, 1996, 52, 4149. CrossRef
24. (a) K. Konno, H. Shirahama, and T. Matsumoto, Tetrahedron Lett., 1983, 24, 939; CrossRef (b) K. Konno, K. Hashimoto, Y. Ohfune, and T. Matsumoto, Tetrahedron Lett., 1986, 27, 607; CrossRef (c) K. Konno, K. Hashimoto, Y. Ohfune, H. Shirahama, and T. Matsumoto, J. Am. Chem. Soc., 1988, 110, 4807. CrossRef
25. K. Hashimoto, M. Horikawa, and H. Shirahama, Tetrahedron Lett., 1990, 31, 7047. CrossRef
26. (a) P. Müller and S. Tohill, Tetrahedron, 2000, 56, 1725; CrossRef (b) H. M. L. Davies, D. G. Stafford, and T. Hansen, Org. Lett., 1999, 1, 233. CrossRef
27. T. Fukuyama, M. Cheung, and T. Kan, Synlett, 1999, 1301. CrossRef
28. T. Sugimura and K. Hagiya, Chem. Lett., 2007, 36, 566. CrossRef
29. T. Higashi, Y. Isobe, H. Ouchi, H. Suzuki, Y. Okazaki, T. Asakawa, T. Furuta, T. Wakimoto, and T. Kan, Org. Lett., 2011, 13, 1089. CrossRef
30. (a) Y. Yokoo, W. Fujii, H. Hori, K. Nagao, Y. Suwa, K. Taniyama, K. Tsuji, T. Yoshida, and H. Nukaya, Alcohol Clin. Exp. Res., 2004, 28, 129S; CrossRef (b) N. Yamaji, Y. Yokoo, T. Iwashita, A. Nemoto, M. Koike, Y. Suwa, T. Wakimoto, K. Tsuji, and H. Nukaya, Alcohol Clin. Exp. Res., 2007, 31, S9. CrossRef
31. A. Stoessl, Tetrahedron Lett., 1966, 21, 2287. CrossRef
32. T. Wakimoto, M. Nitta, T. Chiba, Y. Yiping, K. Tsuji, T. Kan, H. Nukaya, M. Ishiguro, M. Koike, Y. Yokoo, and Y. Suwa, Bioorg. Med. Chem. Lett., 2009, 19, 5905. CrossRef
33. E. D. Myers and R. T. Raines, Angew. Chem. Int. Ed., 2009, 48, 2359. CrossRef
34. H. Saito, H. Oishi, S. Kitagaki, S. Nakamura, M. Anada, and S. Hashimoto, Org. Lett., 2002, 4, 3887. CrossRef
35. T. Wakimoto, K. Miyata, H. Ouchi, T. Asakawa, H. Nukaya, Y. Suwa, and T. Kan, Org. Lett., 2011, 13, 2789. CrossRef