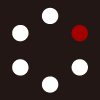
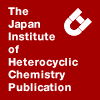
HETEROCYCLES
An International Journal for Reviews and Communications in Heterocyclic ChemistryWeb Edition ISSN: 1881-0942
Published online by The Japan Institute of Heterocyclic Chemistry
e-Journal
Full Text HTML
Received, 18th September, 2015, Accepted, 21st October, 2015, Published online, 28th October, 2015.
DOI: 10.3987/REV-15-826
■ Chemotherapeutic Potential of Acridine Analogs: An Ample Review
Harminder Singh, Harbinder Singh, Sahil Sharma,* and Preet Mohinder Singh Bedi
Department of Pharmaceutical Sciences, Guru Nanak Dev University, Amritsar 143005, Punjab, India
Abstract
Acridine, a novel and pharmacologically important nitrogen containing heterocyclic ring exhibits a variety of potential biological activities. Among the reported therapeutic attributes of acridines, anticancer and antimicrobial are the most comprehensively studied ones. This fused heterocyclic ring has been evidenced in a number of research papers and patents. The principal target of these agents being nucleic acids, to which they bind reversibly by intercalation. This review article is merely an assemblage of all the patents and research work that has been done so far in the recent past on various acridine analogs as chemotherapeutic (anticancer and antimicrobial) agents. The promising activity revealed by these compounds chains their use and places them ahead as reasonably potential drug candidates for the upcoming studies. The present article can be reasonably constructive for those engrossed in the area of hunt for new and effective antimicrobial and anticancer compounds involving acridine as a crucial and central nucleus.CONTENTS
1. Introduction
2. Acridine analogs as anticancer agents
2-1. 9-Substituted acridine analogs
2-2. Bis acridine analogs
2-3. Metal complexes of acridine
2-4. Cyclized acridine analogs
2-5. Acridine hybrids
2-6. Miscellaneous acridine analogs
3. Patents on acridine analogs as anticancer agents
3-1. Patent mapping
3-2. Patent review
4. Acridine analogs as antimicrobial agents
4-1. 9-Substituted acridine analogs
4-2. Cyclized acridine analogs
4-3. Metal complexes of acridine analogs
4-4. Miscellaneous acridine analogs
5. Patents on acridine analogs as antimicrobial agents
5-1. Patent mapping
5-2. Patent review
6. General synthetic protocols for acridines
7. Aspects for future study
1. INTRODUCTION
Heterocycles constitute an important class of compounds and make up for more than 50% of the organic compounds. There are numerous biologically active fused heterocyclic rings that are associated with several biological activities. Nitrogen containing heterocyclic compounds are quite common in animal and plant cells1 and these have shown a prominent role in biological functions viz. bioenergetic transport agents.2 The various substituents in the basic structure, i.e. alterations in the chemical structure of N-heterocyclic compounds determine their pharmacological action, almost all kinds of biochemical properties and thus influence their biological properties in one or other way.
One of the important N-containing heterocyclic compounds being acridine, well known as dibenzopyridine or 10-azaanthracene, is an alkaloid derived from anthracene with an irritating odor. It is characterized by its irritating action on the skin and blue fluorescence showed by solutions of its salts.3 Since their first discovery in the 1880s, acridines have confirmed a broad spectrum of pharmacological properties.4 These were first employed as antibacterial agents during the beginning of the twentieth century,5 and they have rapidly revealed interesting antiproliferative activities against both protozoa6 and tumor cells.
Thus it is quite clear that acridine (structural edifice block) based chemotherapeutic agents have a long history and these have attracted considerable attention due to their potential pharmacological activity and have shown a wide spectrum of various biological activities. It is present in many biologically important molecules, such as amsacrine (1) (cytotoxic and antiviral agents), botiacrine (2) (antiparkinsonian drug), clomacran (3) (tranquilizer), monometacrine (4) (antidepressant), acridine carboxamide (DACA) (5) (antitumor) (Figure 1).7
Previous studies aimed at the development of potential antimicrobial agents derived from acridine nucleus as this nucleus first originated as an antibacterial agent only. Various researchers developed derivatives of acridine that exhibited antimicrobial potential. In the early 90’s, a lot of work was done on the development of new acridine derivatives with antibacterial, antifungal and related activities. Soon after this development, a decline was seen in the same approach as the interest of researchers bowed towards developing acridine analogues as efficient agents to treat cancer. Thus there are more acridine anticancer analogs rather than acridine antimicrobial compounds.
It is quite clear from these researches that acridine-based pharmacophores have wide applications in antimicrobial and anticancer therapy. The primary targets of these agents are nucleic acids, to which they bind through intercalation. There are various factors determining the nucleic acid binding properties of acridines thus determining their chemotherapeutic efficacy and these depend on the nature and positioning of the residues attached to the planar chromophore, some of which are global affinity, geometry of the intercalated complex and drug dissociation rates.8 Moreover the fluorescent properties of acridine derived compounds allow the use of confocal microscopy to examine the accumulation and cellular location of these drug hybrids.9 Therefore, increasing the binding affinity between agents and nucleic acid and retaining the agent–nucleic acid bound form are the two hypothesized rational designs to increase the sensitivity of killing the cancer cells.
Though acridine moiety, as a whole, is an active moiety in almost all types of biological activities but the two discussed here are the most widely studied and effective biological properties. Chemical modification of bioactive components is one of the most common approaches in drug discovery with improved therapeutic effect and consequently methods that successfully minimize their side effects are the focus of much attention.10,11 Thus there was a need of an article that that would describe the potential of acridine analogs and, thus, the present article is a collection of various research works that support the use of differently substituted acridine derived molecules as potent anticancer and antimicrobial agents. The work also presents the patents on such derivatives effective as the above mentioned pharmacological activities. This article would surely help the researchers aiming at the development/study of acridine analogues as either antimicrobial or anticancer compounds.
2. ACRIDINE ANALOGS AS ANTICANCER AGENTS
2-1. 9-Substituted acridine analogs
Dzierzbicka et al. synthesized conjugates of muramyl dipeptide, normuramyl dipeptide, and desmuramyl peptides with acridine/acridone derivatives and evaluated their anticancer activity. The preliminary screening data indicate that the few analogues exhibited potent in vitro cytotoxic activity against a panel of human cell lines with LC50 ranging from 4.10-5.80 M. Analogue 6 (Figure 2) showed an immune stimulating effect on the cytotoxic activity of the NK cells obtained from the spleen of healthy and Ab melanoma bearing animals.12
Delcros et al. reported the effect of spermidine conjugation on the cytotoxic and transport properties of aminoacridines. In a whole cell assay with L1210 and Chinese hamster ovary (CHO) cells, compounds 7, 8, 9 (Figure 2) were the most potent. As determined from competitive uptake studies with [14C] spermidine, all conjugates had a high affinity for the polyamine transport system (PTS), with an excess of spermidine and on the differential effect on cell growth and accumulation in CHO and in the mutant PTS deficient CHO-MG cells and the accumulation of the conjugates through the PTS was found to be poor but still more efficient for the aminoacridines.13
Harrison et al. synthesized tri substituted acridine derivatives as selective telomerase inhibitors. Substitution on the acridine ring at the 2,6,9; 2,7,9; and 3,6,9 positions were done. The interactions of compounds with a parallel quadruplex DNA structure were simulated by molecular modeling methods. The calculated interaction energies were compared with telomerase activity and showed generally consistent correlations between quadruplex affinity and telomerase inhibition. Compounds 10 and 11 (Figure 2) both have elevated duplex affinities compared to other tri substituted compounds, although there was no change in their patterns of IC50 values. The EC50 values as calculated of telomerase inhibition for both these compounds was 0.018 μM each.14
Sondhi et al. synthesized various derivatives with 9-chloroacridines on condensation with sulfadiazine, sulfathiazole, and sulfaacetamide and evaluated for anti-inflammatory, analgesic and kinase inhibition activities. Anti-inflammatory and analgesic activity of all derivatives was carried out using carrageen in induced paw oedema and phenylquinone writhing assay. Compounds 12, 13, 14 exhibited good anti-inflammatory activity and 15, 16 exhibited good analgesic activity (Figure 2). However, these compounds did not showed any kinase inhibition activity.15
Bacherikov et al. synthesized the alkylating N-mustard residue linked to the C-3’ or C-4’ position of the anilino ring with an O-ethylene spacer and evaluated for cytotoxicity against human lymphoblastic leukemia cells (CCRF-CEM) in culture. The results showed that all these new compounds exhibited potent cytotoxicity with IC50 values ranging from 0.002 to 0.7 μM. Compound 17 (Figure 2) demonstrated most potent antitumor effect against A-549 and HCT-116 cell lines.16
Santelli-Rouvier et al. synthesized 9-acridinyl sulfur derivatives viz sulfides, sulfoxides and sulfones and evaluated their anticancer potential on tumor cells. Among 23 compounds prepared, 19 were tested in vitro against the human cancer cell line panel of NCI screening. Compound with epoxy ether 18 (Figure 2) showed the best efficacy on 41 strains within approximately 60 cell lines with GI50 value less than 1.65 μM.17
Delmas et al. synthesized (1,3-benzothiazol-2-yl)amino-9-(10H)-acridinone derivatives via a procedure based on Ullman reaction and evaluated their anticancer potential. Amino-9-(10H)-acridinone compounds 19, 20, 21, 22 (Figure 2) exhibited the highest antiproliferative property and toxicity towards monocytes with IC50 values of 12.5 μM, 14.3 μM, 36.8 μM and 18.2 μM, respectively.18
Goodell et al. synthesized acridine and acridone derivatives and evaluated their topoisomerase inhibiting activity. Compounds with potent activity against herpes simplex virus type-1 (HSV-1) and/or (HSV-2) were further assayed for inhibition of topoisomerase activity to gain insight into the mechanism of action. Their anticancer potential was also examined by assessing their cytotoxicity against vero cells. Compounds 23, 24, 25 and 26 (Figure 2) showed most potent topoisomerase-II inhibition in the micromolar range of 80-100 μM.19
Su et al. synthesized 9-anilinoacridines and acridines bearing an alkylating N-mustard residue, as antitumor agents. It revealed that all newly synthesized compounds were very potent cytotoxic agents against human leukemia and various solid tumors in vitro. Compounds 27 and 28 (Figure 2) were shown to have potent antitumor activity (IC50 values = 0.0281 μM and 0.0056 μM, respectively) in nude mice bearing the human breast carcinoma MX-1 xenograft with 96% and 77% antitumor effect, respectively. The therapeutic efficacies of these two agents were comparable to that of taxol.20
Martins et al. synthesized a new series of benzylamino-substituted acridine derivatives as telomerase inhibitors. Replacement of the previously reported anilino substituents by benzylamino groups resulted in enhanced quadruplex interaction, and for one compound, superior telomerase inhibitory activity. Compound 29 (Figure 2) showed the most potent activity as telomerase inhibitor with a TRAP, EC50 value of 0.03 μM.21
Kapuriya et al. synthesized a series of nitrogen mustard linked to 9-anilinoacridines via urea linkage and evaluated them for antitumor activity. The antitumor studies revealed that these agents exhibited potent cytotoxicity in vitro without cross-resistance to taxol or vinblastine and showed potent antitumor efficacy in nude mice against human tumor xenografts. Compound 30 (Figure 2) was found to be the most cytotoxic against human non-small lung cancer H1299 cell line with IC50 value of 0.03 μM.22
Goodell et al. synthesized acridine based agents and topoisomerase II activity inhibiting pancreatic cancer cell proliferation and apoptosis was studied. The results indicated that the compounds inhibited cell proliferation by inducing a G1-S phase arrest. A model is also developed that explained the molecular basis of inhibition through a DNA “threading” mechanism. The drug-DNA complex was formed that blocked topoisomerase II binding and activity that lead to catalytic inhibition of the enzyme and the induction of apoptosis and programmed cell death. Compound 31 (Figure 2) was active in all three cells lines (MiaPaCa, Su86.86 and BXPC3) with an IC50 value of 1.7 μM.23
Howell et al. synthesized a series of 9-aminoacridine-3- and 4-carboxamides, efficiently using the benzyl/azide click chemistry. These products bind to duplex DNA and have antitumor activity in the HL60 cell line. Compound 32 (Figure 2) revealed most potent activity against HL60 with an IC50 of 0.72 μM.24
Galvez-Peralta et al. synthesized a series of substituted 9-aminoacridines and evaluated them as cytotoxic agents. Compound 33 (Figure 2) diminishes DNA synthesis effectively to stabilize topoisomerase I-DNA adduct formation in intact cells. RNA interference experiment indicated that compound may be non-lethal poison of topoisomerase I. This compound was also found most effective against MEL cell line with an EC50 of 14.8 μM.25
El-Sabaghh et al. synthesized new acridines and hydrazones derived from cyclic β-diketone and evaluated their anticancer and antiviral potential. Most acyclic derivatives especially semicarbazide, hydrazide and thiosemicarbazide showed higher in vitro cytotoxic activity against hepatoma cell line (HepG2) than the cyclized acridine derivatives. The antiviral activity of the new compounds against hepatitis A virus (HAV) using the plague infection reduction assay revealed that the acridine 34 (Figure 2) was the most active one.26
Castillo-Gonzalez et al. synthesized acridine derivatives and evaluated them for their telomerase inhibitory activity. A linear discriminant model was developed to classify a data set of 90 acridinie derivatives. The results of this study suggested that the compound 35 (Figure 2) showed the most potent activity with an IC50 value of 0.03 μM against A2780 ovarian carcinoma cell line.27
Sathish et al. synthesized a series of new 1,3-dimethylacridone derivatives with alkyl side chain (propyl and butyl) with highly basic amine groups at its terminal end, at N10-position and screened them for their cytotoxic activity against human breast adenocarcinoma (MCF-7) and human promyelocytic leukemia (HL-60) cell lines. DNA binding constants (Ki) of selected compounds were also determined using calf-thymus DNA. Results showed that few molecules exhibited good cytotoxic activity with IC50 value less than 10 μM. Compound 36 (Figure 2) having (β-hydroxyethyl)piperazine butyl side chain exhibited most potent cytotoxic activity against MCF-7 cell line and DNA-intercalating properties. Examination of the relationship between lipophilicity and acridone derivatives showed poor correlation.28
Sondhi et al. synthesized various acridine derivatives and evaluated their anti-inflammatory and anticancer activity. Compounds screened for anti-inflammatory activity exhibited 41.17% activity which is better than standard which showed 39% (at 50 mg/kg p.o.) activity. Anticancer evaluation of compounds was carried out against a small panel of human cancer cell lines and compounds 37, 38 and 39 (Figure 2) exhibited good anticancer activity against 7 cancer cell lines MCF-7, HEP-2, COLO-205, 502713, HCT-15, A-549 and IMR-32 cancer at a concentration of 1 × 10-5 M.29
Patel et al. synthesized 4-(acridine-9-ylmethyl)-2H-(substituted chromen)-2-one and evaluated them for their anticancer activity (HL-60, Hep-2 & HEK293T) using MTT assay. Compound 40 (Figure 2) showed good cytotoxicity with IC50 value of 27.53 μM against HL60 cell line.30
El-Sabbagh et al. synthesized new nonclassical acridines and evaluated their cytotoxic activity against liver carcinoma cells (HepG2) using MTT cell viability assay. Decahydroacridin-1,8-dione bearing a 4-methylphenyl moiety 41(Figure 2) showed highest cytotoxic effect with IC50 value 4.42 μg/mL.31
Gao et al. synthesized 2-amino-10-(3,5-dimethoxy)benzyl-9(10H)-acridinone derivatives and evaluated them as antiproliferative agents. Compounds 42 bearing pyrrolidine substituent and 43 with methyl ammonium side chain displayed high cytotoxicity to CCRF-CEM cells and solid tumor cells with IC50 value of 1.7 μM each. Furthermore, compounds 42 and 43 (Figure 2) had strong binding to calf thymus DNA (ct DNA), as detected by UV absorption and fluorescence quenching assays, but limited inhibitory activity towards human topoisomerase 1.32
Paulikova et al. synthesized new acridine-thiazolidinone derivatives and their interactions with calf thymus DNA and cytotoxicity against number of cell lines was studied. Compound 44 (Figure 2) showed highest activity in cytotoxicity study with an IC50 value of 1.3 μM against HL60 cell line. This compound inhibited proliferation of the cells and induced an arrest of the cell cycle and cell death. Its influence upon cells was associated with its reactivity towards thiols and DNA binding activity.33
Alvala et al. synthesized novel acridinedione derivatives and evaluated their tumor cell growth inhibition. New scaffold was discovered as a hSIRT1 inhibitor through virtual screening of in-house database. Based on these hits, a library of compounds was designed of which the most potent compound 45 (Figure 2) showed significant inhibition of SIRT1 activity with an IC50 value of 0.25 μM and also showed a dose dependent increase in acetylation of p53K382 and decrease in SIRT1.34
Kalirajan et al. synthesized some novel oxazine substituted 9-anilinoacridine derivatives and evaluated their antioxidant and anticancer activity as topoisomerase П inhibitors, against Dalton’s lymphoma ascites (DLA) cell growth by in vitro method. The docking studies of the synthesized compounds were also performed on topoisomerase II (1QZR) by using Schrödinger Maestro 9.2 version. The oxazine substituted 9-anilinoacridine derivatives 46-49 (Figure 2) had significant anticancer activity as topoisomerase II inhibitors with an IC50value of 28.02 μg/mL, 26.03 μg/mL, 24.02 μg/mL and 28.01 μg/mL, respectively.35
Lang et al. synthesized acridine derivatives and explored them as DNA-binding anticancer agents. Compound 50 (Figure 2) confirmed DNA-binding capability and topoisomerase I inhibitory activity, also induced apoptosis through mitochondria-dependent intrinsic pathways in K562 cell lines with an IC50 value of 0.54 μM.36
2-2. Bis acridine analogs
Brana et al. synthesized a series of bis{[(9-oxo-9,10-dihydroacridine-4-carbonyl)- amino]alkyl}alkylamines and studied their antiproliferative properties against HT-29 cell lines. Compounds 51 and 52 (Figure 3) showed an interesting cytotoxic profile as they exhibited an IC50 value of 5.3 × 10-7 and 4.0 × 10-7 M, respectively.37
Antonini et al. synthesized bis(acridine-4-carboxamides) and evaluated their anticancer activity. To enhance the outstanding biological response two classes of bis-acridine-4-carboxamides, with a linker between the 4,4’ positions and 1,1’ positions, have been prepared as DNA-binding and potential antitumor agents. The noncovalent DNA-binding properties of these compounds have been examined using gel-electrophoresis and fluorometric techniques. Compounds 53-56 (Figure 3) have most potent cytotoxic activity having IC50 values of 0.057 μM, 0.0020 μM, 0.00043 μM and 0.0001μM, respectively against HT-29 cell line.38
Antonini et al. synthesized two new series of bis acridine derivatives: the bis(pyrimidoacridines) and the bis(pyrazoloacridinecarboxamides) as anticancer compounds. The noncovalent DNA binding properties of these compounds have been examined using fluorometric techniques. In vitro cytotoxic potency of these derivatives toward the human colon adenocarcinoma cell line (HT29) was also evaluated and the compound 57 (Figure 3) was found to be the most potent having IC50 value of 3 nM.39
Yang et al. synthesized mono and dinuclear isoquinolino[4,5-bc]acridine derivatives and evaluated their DNA-binding affinities and cytotoxic activities. Analog 58 (IC50 = 0.025 μM) exhibited the highest in vitro antitumor activity against human lung cancer cell (A549) and 59 (IC50 = 0.246 μM) was the most active one against murine leukemia cell (P388) (Figure 3). DNA binding constant and molecular model indicated that both the length of linker chain and the distance of interchromophore were key impact factors for DNA binding affinity and biological activity. Compound 58 with the long enough linker could exhibit the higher DNA affinity, which contributed to its higher antitumor activity.40
Wang et al. synthesized a series of N-substituted triamine-linked acridine dimers and evaluated their in vivo and in vitro cytotoxicity. These acridine dimers have demonstrated a narrow safe margin, but higher maximum tolerated dose (MTD) in comparison with that of adriamycin in ICR mice. The acridine dimers also demonstrated various anit-COLO 205 solid tumor activities in vivo. Compound 60 (Figure 3) has shown the most significant potency in MTT assay and its TGI is 80% in SCID mice.41
Liu et al. synthesized macrocyclic polyamines bearing mono- or bis- acridine moieties and evaluated their anticancer potential by their DNA interactions. The derivatives have been systematically investigated by UV absorption, fluorescence titration, viscosity measurement, DNA melting and gel electrophoresis experiments. The results showed that bis-acridine 61 (Figure 3) has higher DNA binding ability than that of mono-acridine.42
Pitta et al. synthesized a series of eight new 3-acridin-9-ylmethyl-thiazolidine-2,4-dione and 3-acridin-9-ylmethyl-5-arylidene-thiazolidine-2,4-dione derivatives and evaluated their cell antiproliferation activity using 3-(4,5-dimethyl-2-thiozolyl)-2,5-diphenyl-2H-tetrazolium bromide, MTT assay. Compound 62 (Figure 3) showed the most potent activity against HCT-8 with an IC50 value of 4.45 μg/mL.43
2-3. Metal complexes of acridine
Augustus et al. synthesized bis(acridine)platinum(II) complexes. The 4+ charged conjugates associated strongly with double-stranded native DNA (Ki > 106), possibly through bisintercalation. A cell viability assay was used to demonstrate that both compounds are capable of mediating cytotoxicity at micromolar concentrations in SNB19 brain tumor cells. Complex 63 (Figure 4) showed approximately 5 folds more affinity than others.44
Guddneppanavar et al. incorporated diamine nonleaving group in platinum-acridinylthiourea. The DNA sequence specificity of the conjugates and their antiproliferative potential in HL-60 and H460 cells were investigated. Conjugate 64 (Figure 4) showed the strongest non-cisplatin-type DNA damage in polymerase stop assays and superior cell kill efficacy with an IC50 value of 70 nM in H460 lung cancer.45
Ma et al. synthesized thiourea- and guanidine-modified acridine-4-carboxamides and a corresponding platinum-intercalator conjugate and evaluated them as cytotoxic agents. Compound 65 (Figure 4) among the newly synthesized compounds was found to be the most active in NCI-H460 cells with an IC50 value of 2.4 μM carrying potential for the treatment of chemoresistant tumors.7
Choudhury et al. synthesized acridine platinum complexes and evaluated their cytotoxic activity. Circular dichroism spectra and chemical foot printing experiments suggest that 66 (Figure 4), the most active derivative produces a structurally severely perturbed DNA with features of a Hoogsteen base-paired biopolymer. This active derivative was tested for its cytotoxicity in human leukemia (HL-60) cell line displayed an IC50 value of 0.65 μM.46
Eiter et al. synthesized gold (I) analogues of a platinum-acridine and evaluated their antitumor and antituberclosis activity. Unlike platinum, gold does not form permanent adducts with DNA, and its complexes are less cytotoxic in nonsmall-cell lung cancer cells. Complex 67 (Figure 4) was found to be slightly more cytotoxic than other acridine complexes. A similar effect was observed in the human leukemia cell line where it exhibited an IC50 value of 1.76 μM.47
Ma et al. synthesized platinum-acridine derivatives by replacement of a thiourea-S with an amidine-NH donor group. The comparative kinetic study of amidine modified complex and thiourea-based compound 68 (Figure 4) showed that the replacement of the sulfur with an imino donor group reduced the metal’s reactivity with cysteine sulfur but enhances its binding with DNA nitrogen. The cytotoxicity of this compound was established by studying its activity in non small cell lung cancer, where its IC50 value was found to be 70 nM.48
Graham et al. synthesized carboxylic acid ester-functionalized platinum-acridine hybrid molecules as anticancer agents. In-line high-performance liquid chromatography and electrospray mass spectrometry (LC-ESMS) showed that the ester moieties undergo platinum-mediated hydrolysis in a chloride concentration-dependent manner to form carboxylate chelates. Complex 69 (Figure 4) was the most active with an IC50 value of 11 nM in NCI-H460 cell line and potential applications of the chloride-sensitive ester 70 hydrolysis as a self-immolative release mechanism for tumor-selective delivery of platinum-acridines were also reported.49
Ding et al. synthesized DNA-targeted platinum-containing hybrid as anticancer agents based on metal-mediated amine-to-nitrile addition. Product mixtures were quantitatively analyzed by automated in line high performance liquid chromatography-electrospray mass spectrometry (LC-ESMS) analysis and subjected to cell viability screening using a nonradioactive cell proliferation assay. Compound 71 (Figure 4) exhibited the most potent activity with an IC50 value of 2.8 nM against NCI-H460 cells.50
2-4. Cyclized acridine analogs
Antonini et al. synthesized N-5,2-di(ω-aminoalkyl)-2,6-dihydropyrazolo[3,4,5-kl]acridine-5- carboxamides. The non covalent DNA-binding properties of these compounds have been examined using a fluorometric technique. Two highly DNA-affinic and potent cytotoxic compounds 72 and 73 (Figure 5) have been identified as new leads in the antitumor strategies and the in vitro cytotoxic potency of these derivatives against human colon adenocarcinoma cell line (HT29) was evaluated and IC50 values of these most potent compounds (72 and 73) was found to be 0.0039 μM and 0.0037 μM, respectively.51
Delfourne et al. synthesized various analogues of meridine and evaluated its antitumor activity. The IC50 value of these 25 compounds ranged over 5 log concentrations and the compounds 74-77 (Figure 5) exhibited significantly higher in vitro antitumor activity than meridine with IC50 range from 0.0001-0.01 μM against a panel of 12 different cell lines (PC-3, A427, A549, T-47D, MCF7, HCT-15, LoVo, J82, T24, SW1088, U-373MG, U-87MG). These compounds were subjected to further pharmacological investigation including in vivo testing on both conventional murine tumors and human tumors grafted onto nude mice.52
Gamage et al. establishedstructure-activity relationships for pyrido-, imidazo-, pyrazolo-, pyrazino-, and pyrrolophenazinecarboxamides as topoisomerase targeted anticancer agents. Compounds with a fused five-membered heterocyclic ring were generally less potent than the pyrido[4,3-a]phenazines. Compound 78 (4-methoxypyrido[4,3-a]phenazine) (Figure 5) was the most potent one with an IC50 value of 2.4 nM, gave modest growth delays in H69/P xenografts with oral dosing.53
Delfourne et al. synthesized novel ring D analogues of the marine pyridoacridine ascididemine and evaluated their anticancer potential. The compounds and ascididemine used as the control were tested at six different concentrations on 12 different human cancer cell lines. Compound 79 (Figure 5) was the most potent for the antitumor activity (determined in vitro) with an IC50 value of 7 nM against U-373MG and tolerability (determined in vivo) with MTD values 160 mg/kg/day.54
Antonini et al. synthesized DNA-binding agents with potential antitumor activities bearing two cationic side chains. In vitro cytotoxic properties of these derivatives against three human colon adenocarcinoma cell lines and against some cell lines of the NCI panel were also reported. The mechanism and efficiency of noncovalent DNA binding of these compounds were examined using gel electrophoresis and fluorometric techniques. Compound 80 (Figure 5) showed the most potent activity with an IC50 value of 0.04 nM on HT29 cell line.55
Bu et al. synthesized pyrazolo[3,4,5-kl]acridine and indolo[2,3-a]acridine and evaluated as anticancer agents. Compound 81 (Figure 5) was found to be appreciably cytotoxic against a panel of cell lines (P388, LLTC, JLC, JLA, JLD).56
Antonini et al. synthesized a series of thiadiazinoacridines and evaluated the same as antitumor agents. In vitro cytotoxic potencies of these derivatives toward six tumor cell lines were reported. In vivo antitumor activity of some selected derivatives, endowed with relevant cytotoxic activity against murine leukemia P388 was reported, and compound 82 (Figure 5) was the most effective one with an IC50 value of 0.027 μM and thus it was identified as a new lead in the development of anticancer tetracyclic acridine derivatives.57
Cookson et al. synthesized pentacyclic acridinium salts and evaluated for destabilizinng telomeric integrity. Stability in tissue culture media in the presence of A549 lung and MCF-7 breast tumor cells, metabolic stability when incubated with rat liver microsomes, and rate of uptake and subcellular location in A549 and MCF-7 cells was done. Compound 83 (Figure 5) exhibited the most favorable pharmaceutical profile, mean GI50 and SI value of 13.18 μM.58
Sanchez et al. synthesized a series of new acridines by cyclodehydration of N-(2,3-dihydro-1,4-benzodioxin-6-yl)anthranilic acid in acidic media. Several of the studied compounds displayed significant cytotoxic activity. Pentacyclic analogues 84 and 85 (Figure 5) were more cytotoxic with IC50 value in the range of 0.4-0.8 μM and 0.5-0.9 μM, respectively against L1210 and HT-29 cells, compared to tetracyclic compounds.59
Cheng et al. synthesized polycyclic acridines and evaluated their biological activities against tumor cell line. Growth inhibition potency in the NCI60 human tumor cell line panel is more marked in compounds with greater DNA duplex binding affinity. Compounds 86 and 87 (Figure 5) have few notable properties. They have low growth-inhibitory activities in the NCI60 human tumor cell panel with GI50 value of 12 μM ans 81.3 μM, having potent telomerase inhibitory activity inferred from the TRAP assay TRAPIC50 < 0.5 µM, selectivity index of 32 and 195, respectively and showed the greatest differential binding affinities between quadruplex and duplex DNA with K being 2.4 × 107 and 2.5 × 107 M-1, 0.038 × 107 and 0.044 × 107 M-1, respectively.60
Roopan et al. synthesized 9-chloro-6,13-dihydro-7-phenyl-5H-indolo[3,2-c]-acridines in the presence of SnO2 nanoparticles under microwave irradiation. Indoloacridines were screened for their in vitro hemolytic activity, on human erythrocytes and cytotoxicity, on HeLa and Vero cells. Compounds 88-90 (Figure 5) were inactive on both cells as their IC50 was more than 50 μg/mL.61
Liao et al. synthesized 12-N-methylated and non-methylated 5,6-dihydrobenzo[c]acridine derivatives. Their interactions with c-MYC quadruplex was evaluated using fluorescence resonance energy transfer (FRET) melting assay, circular dichroism (CD) spectroscopy, surface plasmon resonance (SPR), polymerase chain reaction (PCR) stop assay and molecular modeling. The reverse transcription (RT) PCR assay showed that compound 91 (Figure 5) could down-regulate transcription of c-MYC gene in Ramos cell line (IC50 value of 5 μM) but had no effect in CA46 cell line.62
2-5. Acridine hybrids
Alarcon et al. synthesized acridine-diaminopurine heterodimers by a linker containing an N,N’-substituted guanidine. The interactions of the new heterodimers with a basic site containing oligonucleotide were compared, and their cytotoxicity was measured in the presence or absence of the antitumor alkylating agent. In compound 92 (Figure 6), linker was tethered to position 9 and it showed the most synergistic effect with BCNU (alkylating agent). The synthesized compounds were tested for their cytotoxicity on A549 cell lines where the compound 90 was the most effective one with an IC50 value of 6.5 μM.63
Kamal et al. synthesized C8-linked pyrrolo[2,1-c][1,4]benzodiazepine-acridone/acridine hybrids and evaluated as anticancer agents. Compound 93 (Figure 6) showed the high CT-DNA binding affinity elevating the helix melting temperature of CT-DNA by 12.5 °C after incubation for 18 h and the cytotoxic activity revealed that this compound has the lowest IC50 value of 0.05 μM against renal A498 cancer cell line.64
Jones et al. synthesized artemisinin-acridine hybrids and further evaluated as antitumor and antimalarial agents. These compounds induced apoptosis in HL60 cells, obtained by flow cytometry, at the G1 phase of the cell cycle. Among the series of synthesized compounds, only compound 94 (Figure 6) showed 2-4 folds more potency than DHA against HT29-Ak cell line with an IC50 value of 9.91 μM.65
Malachowska-Ugarte et al. synthesized mycophenolic acid conjugates containing nitro-acridine/acridone derivatives and evaluated their anticancer potential against various cancer cell lines. These conjugates possess different lengths of the linker between MPA and heterocyclic units. All the hybrids exhibited potency with IC50 ranging from 0.108-0.37 µM. Among all the compounds, 95 (Figure 6) was the most potent one with an IC50 value of 0.02 µM against L1210 cancer cell lines.66
2-6. Miscellaneous acridine analogs
Charmantry et al. synthesized 4-hydroxymethyl-3-aminoacridine derivatives and evaluated their anticancer potential. The most cytotoxic molecules were 96 and 97 (Figure 7) with an IC50 value of 0.02 μM (against L1210 cell lines) and 0.04 μM (against HT29 cell lines), respectively. The study revealed that the compound 97 was uniformly distributed both in the cytoplasm and in the nucleus, whereas compound 96 was essentially localized in cytoplasmic granules.67
Asche et al. synthesized 3-amino-4-hydroxymethylacridine derivatives and checked their cytostatic activity. Compound 98 (Figure 7) was the most active molecule but was 80-fold less active than the unsubstituted lead structure on HT29 cells.68
Giorgio et al. synthesized new series of diaminoacridinie derivatives obtained from proflavine and N-(6-amino-3-acridinyl)acetamide and assessed them for their cytotoxic and antileishmanial activities. Among all the compounds tested, compound 99 (Figure 7) exhibited the highest anticancer activity against human monocytes (IC50 value of 0.77 μM) suggesting that acridine compounds could interact with mammalian and protozoan cells by different mechanisms.69
Fadeyi et al. synthesized a novel series of fluorinated acridones from 5-trifluoromethyl-1,3- cyclohexanedione. The cytotoxic activities of the compounds were studied in several cancer cells. Compound 100 (Figure 7) was appreciably active covering a wide range of cancer cell lines and exhibiting an IC50 value in the range of 0.13-26 μM.8
Benchabane et al. synthesized 3,6-disubstituted acridines by acylation of proflavine and evaluated their photo-inducible cytotoxic and clastogenic activity. The cytotoxicity and photo-enhanced cytotoxicity were evaluated on both tumor CHO cells and human normal keratinocytes and compared their corresponding clastogenicity by the micronucleus assay. Compounds 101 and 102 (Figure 7), on the contrary, displayed a strong clastogenicity with MCC 1.15, 0.03 µM and 1.01, 0.007 µM (-ve light, +ve light), respectively.70
Janovec et al. synthesized 3,6-bis((imidazolidinone)imino)acridine derivatives and evaluated them as cytotoxic agents. The ctDNA-binding (calf-thymus) was examined by UV-vis, fluorescence and CD spectroscopy. The highest activity in cytotoxic tests was found for 103 (Figure 7) exhibiting an IC50 value of 2.12 μM against HL60 cancer cell lines. Molecular dynamics and solvent-accessible surface area calculations indicated that the decrease of K with alkyl extension is due to negative entropic change upon binding.71
Ankers et al. synthesized novel derivative of the anti-tumor agent N-[2(dimethylamino)ethyl]- acridine-4-carboxamide (DACA). An in vitro transcription assay revealed that formaldehyde-mediated C4-DACA-DNA adduct 104 (Figure 7) selectively formed a CpG and CpA dinucleotide sequences, which was strikingly similar to that of formaldehyde-activated anthracenediones such as pixantrone.72
3. PATENTS ON ACRIDINE ANALOGS AS ANTICANCER AGENTS
3-1. Patent mapping (Table 1)
3-2. Patent review
Atwell et al. filed a patent on substituted o-phenylenediamineacridine compounds having antitumor activity in 1983. In this study a series of o-phenylenediamine derivatives were synthesized because 3-methylamino, 3-NH2 had more activity than unsubsituted or substituted-AMSA. All synthesized derivatives were evaluated in vitro and vivo for anticancer activty and all compounds showed good antitumor profile. Compounds 105-107 (Figure 8) exhibited the appreciable antitumor effect against lung cancer cells exhibiting IC50 value in micromolar range.73
Watanabe et al. filed a patent on potential anticancer agents derived from acridine in 1991. All synthesized compounds were tested against the growth of mouse leukemia L1210 cells. Compound 108 (Figure 8) was the most potent one against L1210 cell lines with an IC50 value of 2.3 × 10-11 μM. The invention revealed that the basic and planar molecules readily intercalate into DNA and lead to damage of DNA functions permanently.74
Baguley et al. filed a patent on acridine carboxamides for the treatment of cancer in 1993. A novel treatment schedule for administration of N-[2(dimethylamino)ethyl]acridine-4-carboxamide anticancer drug was introduced in which the drug was administered in a divided-dose schedule comprising two or more administrations at frequent intervals was adopted. The continuous drug exposure in a variety of human and mouse cell lines and in a panel of 60 human cell lines was studied and the compound 109 (Figure 8) was appreciably potent against almost all cell lines exhibiting IC50 value in micromolar range.75
Denny et al. filed a patent on bis(acridinecarboxamide) and bis(phenazinecarboxamide) as antitumor agents in 1997. All the derivatives were evaluated against Lewis lung carcinoma cell line (LLTC), human leukemia line (JLc), murine leukemia (P388) for anticancer evaluation. Compound 110 (Figure 8) exhibited most appreciable antitumor profile against all the cell lines with an IC50 ranging from 7-28 nM.76
Gribble et al. filed a patent on bis(9-aminoacridine) DNA intercalating agents having antitumor activity in 1998. Bisacridine derivatives were synthesized by using various configurations of linkers and in vitro anticancer activity was studied. In compound 111 (Figure 8) cis-cis configuration of linker showed the most potent activity against L1210 murine leukemia cells with an ID50 value of 0.44 μM.77
Antonini et al. filed a patent on bis acridinecarboxamides having antitumor activity in 1998. Compounds were synthesized in dipolar aprotic solvent by using copper and further cyclization was done in the presence of polyphoshoric acid and synthesized compounds were evaluated for anticancer activity against human HT 29 cell line. Compounds 112 and 113 (Figure 8) were the most effective ones among the series with an IC50 value of 0.0001 μg/mL each.78
Han et al. filed a patent on anti-cancer composition in 1999. In this study acridine derivatives and guanosine compounds were used in different ratios by their weights. Acriflavine 114 (acridine derivative) and guanosine hydrate 115 (guanosine compound) (Figure 8) were used as anticancer agents. Composition AG-60 (one of the efficient ratio mixture of acriflavine and guanosine hydrate) was found to be the most effective anticancer agent with reduced toxicity in comparison to their anticancer activity, individually.79
Snodin et al. filed a patent in 2006 on cancer treatment using synthesized 3,6,9-subsituted acridines. All the compounds were evaluated for their anticancer potential by various assay techniques including Taq polymerase assay, modified telomeric repeat amplification protocol (TRAP) assay, FRET DNA melting assay and growth inhibition assay. Compound 116 (Figure 8) exhibited the most effective anticancer profile exhibiting an IC50 value of 2.30 μM against ovarian carcinoma cancer cell lines.80
Neidle et al. filed a patent in 2007 on therapeutic acridine and acridone compounds. The invention pertains to pharmaceutical compositions comprising these compounds, and the use of such compounds and compositions, both in vivo and in vitro, to inhibit telomerase, to regulate cell proliferation and in the treatment of proliferative conditions, such as cancer. The most appreciable compounds of the acridine series were 117-119 (Figure 8) with an average IC50 value of 0.02, 0.74 and 0.1 μM against almost all the cancer lines.81,82
Pitta et al. filed a patent on acridine derivaties with antitumoral activity in 2007. More specifically, the invention involves the synthesis of acridine-thiazolidinic derivatives and acridine-imidazolinic derivaties. Compounds 120-122 (Figure 8) were the most appreciably active anticancer agents.83
Su et al. filed a patent in 2008 on 9-anilinoacridine alkylating agents. Alkylating nitrogen mustard derivatives were found to exert cytotoxicity by interstand cross linking of DNA. The cytotoxicity of the compounds of invention was studied using XTT assay and it was found that the compound 123 (Figure 8) was the most active one against A-549 cell line with an IC50 value of 0.0056 μM.84
Bierbach et al. filed a patent on platinum acridine anticancer compounds in 2009. The in-vivo (xenograft model) and in vitro studies (DNA polymerase inhibiton assay and cyotoxicity assay) revealed that out of the compounds synthesized, 124-126 (Figure 8) exhibited the most effective anti-tumor activity with an IC50 value ranging between 0.026-0.028 μM against NCI H-460 cell lines.85-87
Bierbach et al. filed a patent in 2012 on targeted delivery and prodrug design of platinum acridine anticancer compounds. These platinum agents did not induced DNA cross-links unlike as clinical platinum agents. To overcome the problem of tumor resistance (damage DNA radically) novel DNA-targeted hybrid agents were designed. Compound 127 (Figure 8) was the most potent one among the series of compounds synthesized with an IC50 value of 0.008 μM against NCI-H460 cell lines.88
Carvalho et al. filed a patent in 2013 on molecular compound comprised of acridine molecule chemically linked to a carboxylic group which can be used for the selective destruction of solid tumor cells. The invention provides a product comprised of a molecular compound denominated oncoloose, which is selectively targeted to the interior of tumors. Compounds 128-132 (Figure 8) were the most potent anti tumor compounds among the series of compound synthesized in the invention.89
4. ACRIDINE ANALOGS AS ANTIMICROBIAL AGENTS
4-1. 9-Substituted acridine analogs
A series of new 1-nitroacridine derivatives were synthesized and explored as antimicrobial agents by Ngadi et al. All synthesized compounds were screened for the evaluation of antimicrobial properties against Gram positive bacteria, Gram negative bacteria, mycobacteria and fungi. Compounds 134 and 135 (Figure 9) were the most efficient growth inhibitors with MIC value of 50 μg/mL against Escherichia coli, Staphylococcus aureus and Candida albicans, and possessed strong affinity for DNA, which could probably be due to the pharmacophoric group N-(CH2)-N.90
The antimicrobial activity of 9-oxo and 9-thioacridines towards Escherichia coli, Staphylococcus aureus, Mycobacterium smegmatis and Candida albicans using microplate assay was reported by Cremieux et al. The activity against M. smegmatis depends upon the substitution on the aromatic ring; a methoxy substitutent was favored at position 4 of the ring, an amino substituent at position 2 or 3 enhanced the bacteriostatic effect of 9-thioalkylacridines and a dialkyl aminoalkyl side chain increased the activity of 3-amino substituted derivatives. Compounds 136 and 137 (Figure 9) were found to be the most potent with MIC value of 10 μg/mL against M. smegmatis.91
The effect of structure on antibiotic action of new 9-(ethylthio)acridines was highlighted by Nesmerak et al. Six new 9-(ethylthio)acridine derivatives were examined for antibacterial and antifungal activities against 10 bacterial and 8 yeast strains by agar-diffusion method. Compounds 138 and 139 (Figure 9) exhibited maximum inhibitory activity with MIC ranging between 12-128 μg/mL against six bacterial strains.92
Delmas et al. synthesized 1,3-benzothiazol-2-yl)amino-9-(10H)-acridinone derivatives via a procedure based on Ullman reaction and assessed for their in vitro antileishmanial activity. Two derivatives 140 and 141 (Figure 9), revealed a selective index more than 50.18
Drogon et al. synthesized new acridone-4-carboxylic acid derivatives as potential inhibitors of hepatitis C virus infection and tested using the direct fluorometric helicase activity assay to determine the inhibitory properties of the derivatives towards the NS3 helicase of hepatitis C virus (HCV). Compound 142 (Figure 9) was efficient RNA replication inhibitor with selectivity index and IC50 value of 40.5 and 3.8 μM, respectively.93
Patel et al. carried out Bernthsen synthesis of acridine derivatives and evaluated them for antimicrobial activity. Synthesized compounds, 4-(acridine-9-ylmethyl)-2H-(substituted chromen)-2-ones, were screened for their in vitro antibacterial activity against Staphylococcus aureus, Staphylococcus pyogenes, Escherichia coli and Pseudomonas aeruginosa and antifungal activity against Aspergillus niger by agar plate disk diffusion method. All compounds showed moderate antifungal activity whereas compound 143 (Figure 9) exhibited most potent activity against both Gram positive bacteria S. aureus and Gram negative bacteria E. coli.30
Ali et al. synthesized some new 1,3-thiazoles, 1,3,4-thiadiazoles, 1,2,4-triazoles and 1,3-thiazines incorporating acridine and 1,2,3,4-tetrahydroacridine moieties. Synthesized compounds were evaluated in vitro for their antibacterial activities against Staphylococcus aureus, Streptococcus pyogenes, Pseudomonas fluorescens and Pseudomonas phaseolicola and antifungal activity against Fusarium oxysporum and Aspergillus fumigates fungal strains. Compounds 144-147 (Figure 9) were found to possess high inhibition at 2 mg/mL concentration against most microorganisms.94
El-Sabbagh et al. synthesized new non-classical acridines derived from dimedone and evaluated the synthesized compounds for antimicrobial activity. Results of antimicrobial activity revealed that decahydroacridine-1,8-dione 148, bearing a 3-nitrophenyl group was the most active against both Gram-positive and Gram-negative bacteria having highest zone of inhibition. Most potent antifungal activity was shown by 149 (Figure 9) having chloro substituent and increase in number of chloro atoms led to increase in antifungal activity against Candida albicans.31
Singh et al. reported the synthesis and antibacterial activity of benzotriazole substituted acridines. The in vitro antibacterial activity of the synthesized 9-substituted acridine derivatives was assessed against S. aureus, B. subtilis and E. coli by cup-plate method on nutrient agar medium at the most active concentration level of 1000 µg/mL. Compounds 150 and 151 (Figure 9) showed good antibacterial activity, concluding that the substitution at C-2 position of acridine ring either with -OMe or -Me increased the activity.95
Kalirajan et al. explored microwave assisted synthesis for some novel pyrazole substituted 9-anilinoacridine derivatives and evaluated them for antimicrobial activity. The compounds were tested for their antimicrobial activity against Bacillus subtilis, Escherichia coli and Candida albicans by cup-plate method. Compounds 152-154 (Figure 9) exhibited good inhibitory effect against Bacillus subtilis and Escherichia coli at the concentration of 25 μg/mL and Candida albicans at the concentration of 50 μg/mL.96
The synthesis of new fluorescent pyrazolo[4,3-a]acridine derivatives possessing strong antibacterial activity was reported by Daghigh et al. The antibacterial activity of the synthesized compounds was tested against a panel of strains of Staphylococcus aureus, methicillin resistant S. aureus, clinically isolated S. aureus and Bacillus subtilis; Pseudomonas aeruginosa and Escherichia coli species by broth dilution method. All the compounds were found to be super-effective against both Gram positive and Gram negative bacteria with compound 155 (Figure 9) being the most potent among the tested compounds.97
Benoit et al. synthesized a series of 9-alkylaminoacridines and evaluated for activity against two strains of methicillin-resistant and methicillin-sensitive Staphylococcus aureus. Decyl analog 156 (Figure 9) displayed good antibacterial activity with IC50 value of 2.61 μM and showed a very low degree of DNA intercalation.98
4-2. Cyclized acridine analogs
Synthesis of 7, 10, 11, 12-tetrahydrobenzo[c]acridin-8(9H)-one derivatives by microwave irradiation and evaluation for antimicrobial activity against some bacteria and fungi strains by disk-diffusion method and broth dilution assay were reprted by Ngadi et al. Compounds 157-159 (Figure 10) showed good antibacterial and antifungal activity, which might be due to the presence of chlorine and hydroxyl groups in p-position and nitro group in o-position in phenyl ring.99
Rahimizadeh et al. synthesized imidazo[4,5-a]acridones and imidazo[4,5-a]acridines as potential antibacterial agents. Compounds 160, 161 and 162 (Figure 10) showed highest sensitivity (at 50-250 μg/cm in paper disc diffusion method) against Escherichia coli, Staphylococcus aureus, Pseudomonas aeruginosa, and Bacillus subtilis which demonstrated that halogen substituted imidazo[4,5-a]acridines exhibited better activity than others.100
Bontemps et al. isolated pyridoacridine alkaloids from different chromotypes of the ascidian Cystodytes dellechiajei and evaluated the antimicrobial potential of the isolated alkaloids. The antimicrobial activities of extracts and isolated compounds from different color morphs of C. dellechiajei were determined by a liquid growth inhibition assay based on a NCCLS method. Extracts and pure compounds from C. dellechiajei were tested against the two bacterial strains Escherichia coli and Micrococcus luteus. The extracts of blue (163) and purple ascidian color morphs (164, 165, 166, 167, 168) (Figure 10) showed the most potent activity with MIC values ranging from 0.2 to 2.6 µM against E. coli and from 0.3 to 17.4 µM against M. luteus.101
Prabakaran et al. reported the synthesis and antimicrobial activities of nitro substituted indolo[3,2-c]acridines. The synthesized acridines were screened for their antibacterial activity against Gram positive (S. aureus, B. subtilis) and Gram negative (K. pneumonia, S. typhi) bacteria using agar well diffusion method. All the compounds exhibited antibacterial action with compound 169 (Figure 10) being the most potent with MIC value of 35 μg/mL against S. aureus bacteria.102
Konda et al. synthesized some novel acridine derivatives and evaluated them by agar diffusion method against Bacillus subtilis, Proteus vulgaris, Staphylococcus aureus and Escherichia coli. Compound 170 (Figure 10) displayed promising activity against all strains at the concentration of 25 μg/mL.103
4-3. Metal complexes of acridine analogs
Eiter et al. synthesized gold (I) analogues of a platinum-acridine and evaluated for their antituberclosis activity. Complex 171 showed the best antimycobacterial activity with IC50 value less than 0.29 µM, complex 172 proved to be the most Mtb-selective complex with IC50 value of 0.65 µM (Figure 11).47
4-4. Miscellaneous acridine analogs
Carole et al. synthesized 4,5-di-substituted and 4-mono-substituted acridines homologues and compare their in vitro antileishmanial activity. These compounds appeared too cluttered on both sides to involve a strong intercalative binding with DNA. As a consequence, they did not demonstrate strong antiproliferative activity on tumoral cells. Compound 173 (Figure 12) displayed a strong inhibitory activity on the intracellular amastigote selective index with IC50 value of 0.6 μM.104
Ashry et al. reported the synthesis of acridine derivatives from dimedone by microwave irradiation. The antimicrobial activity against Staphylococcus aureus, Candida albicans, Escherichia coli and Pseudomonas aureus was evaluated by agar diffusion technique. The compound 174 (Figure 12) resulted as the potent amongst the acridine derivatives synthesized ascompare to reference drug.105
Giorgio et al. synthesized new series of diaminoacridinie derivatives having antileishmanial activity. Antileishmanial activity was assessed against parasites of Leishmania infantum species. Compounds 175 and 176 (Figure 12) exerted the most specific antileishmanial activities with specificity index (SI) value of 163.8 and 100.0 respectively, suggesting that acridine compounds could interact with mammalian and protozoan cells by different mechanisms.69
5. PATENTS ON ACRIDINE ANALOGS AS ANTIMICROBIAL AGENTS
5-1. Patent mapping (Table 2)
5-2. Patent review
Seneca et al. filed a patent in 1964 on 4-alkyl resorcinolates of amino-acridines as active pathogenicidal agents i.e. chemotherapeutically active fungicidal, bactericidal and trichomonocidal agents, also useful as anthelmintics and in the elimination of other endoparasites, e.g. amoebic infestations. Among the salts of the present invention, 177 (Figure 13) was found to the most potent as it inhibited the growth of all the organisms especially Candida albicans, Bacillus subtilis and Pseudomonas aeruginosa with a dilution of 1:20000.106
In 1973, Cohn et al. filed a patent on compositions containing acridine derivatives for the control of insects, mites, leporine animals and rodents. Compounds 178-183 (Figure 13) were evaluated by Plutellae test and Tetranychus test. The efficacy of the compound was measured by obtaining the degree of destruction. The most active compound of the series showed 100% degree of destruction at a concentration as low as 0.02%.107
Eitan et al. filed a patent on complexes of phosphanilic acid (184) and acridine derivatives (185) as antibacterial agents in 1974 (Figure 13). The evaluation of antibacterial activity was done by agar inclusion method followed by substantivity test. The 1:1 complex was found to be effective against 40 hospital strains of Pseudomonas sp. and various clinical strains of Proteus sp. The drug action was found to be bactericidal.14 This compound was the most potent among the series with an average MIC of 3.1, 5.1 and 6.3 μg/mL against Shigella sp., Pseudomonas sp., and Streptococcus albus, respectively.108
Schulenberg et al. filed a patent in 1979 on aminoalkoxy substituted 9-(aryl or aralkyl)-acridines, useful as trypanosomacidal and antibacterial agents. Evaluation of the synthesized compounds for antimicrobial activity was done in vitro by the conventional serial dilution technique against several species of bacteria and fungi. The potent antimicrobial compounds of the series were 186-188 (Figure 13) with minimum inhibitory concentration (MIC) against Staphylococcus aureus being 15.6, 7.8, 7.8 μg/mL, resepectively.109
Kovacs et al. filed a patent on aminoacridine-α, β-(D)- or -(L)-N-glycoside derivatives, the salts thereof and a process for the preparation of such compounds in 1984. The aminoacridine compounds 189 and 190 (Figure 13) were found to be most efficacious at the concentration of 50 µg/mL against Bacillus subtilis, Salmonella typhymurium, Proteus vulgaris, Escherichia coli, Shigella flexneri etc. whereas the diglucoside of 190 was found to show efficacy against fungi Aspergillus funigatus and Aspergillus niger.110
In 1985, Capps et al. filed a patent on pyrazolo[3,4,5-kl]acridine compositions and methods for their production and use, describing these derivatives as antibacterial and antitumor agents. The evaluation of antimicrobial activity was done by in vitro antibacterial/antifungal (ABF) test. The compounds were tested in an agar-disk diffusion assay and the active compounds of the series were 191-196 (Figure 13) exhibited MIC of < 0.46 μM against the various strains.111
Denys et al. filed a patent on 9,10-dihydroacridine derivatives possessing antimicrobial activity in 1998. All synthesized compounds were evaluated for antimicrobial activity against bacteria (Staphylococcus aureus, Escherichia coli, Proteus vulgaris, Pseudomonas aeruginosa, Bacillus cereus and Bacillus subtilis), fungi (Candida albicans and Trichophyton mentagrophytes) and virus (hepatitis B (HBV) and herpes simplex type 1 (HSV-1)). Compounds 197 and 198 (Figure 13) were found to be most active antimicrobials against the test organisms.112
In 2010, Riscoe et al. filed a patent on acridone compounds as chemosensitizing and antiparasitic agents. The evaluation of antiparasitic action of the synthesized compounds was done by in vitro assessment of intrinsic antimalarial activity against the chloroquine sensitive D6 and Dd2 strains of P. falciparum. The most potent compound 199 showed IC50 value of 12 nM against D6 strain and 19 nM against Dd2 strain. In addition, the evaluation of in vitro antimalarial activity showed compound 200 as the most potent one with IC50 value of 17 nM and 20 nM against D6 and Dd2 strains of P. falciparum, respectively (Figure 13).113
In 2011, the compounds exhibiting antimicrobial activity were reported in a patent filed by Lavoie et al. The present invention includes synthesis and evaluation of many classes of compounds, in which 2-substituted-3,4,9,10-tetramethoxybenzo[a]acridines (201) and their 7-methylbenzo[a]acridinium derivatives (202) (Figure 13) were found to be potent in the treatment of bacterial infections including infections by Gram-positive bacterial strains, Gram-negative bacterial strains and multiple drug-resistant bacterial strain.114
6. GENERAL SYNTHETIC PROTOCOLS FOR ACRIDINES (Figure 14)
7. ASPECTS FOR FUTURE STUDY
In short, we summarized the importance and need of acridine analogs as quite powerful anticancer and antimicrobial compounds. Studies suggested that the use of various substituted derivatives of acridine have left a landmark in the field of search for new anticancer agents. The acridine class of compounds, as a whole, has been explored using different substituents viz. substitution at 9th position, metal complexes of acridine and hybrids of the same. As the substitutions determine the binding of the molecule, thus, these affect their efficacy in terms of pharmacological properties.
The reports suggested that among the various substitutions made on the acridine ring, the 9-substituted acridines, the metal complexes and the hybrids of acridines have shown the most potent and effective anticancer profile. The IC50 values of the synthesized compounds by various researchers were found to be as low as in micromolar range, therefore supporting the use of these analogs as antitumor agents. For instance, 9-substituted acridine derivatives synthesized by Harrison et al.,14 Kamal et al.,64 Su et al.,20 and Castillo-Gonzalez et al.,27 exhibited IC50 values of 0.018 μM, 0.01 μM, 0.0281 μM and 0.03 μM, respectively. Various metal complexes of acridines synthesized by Choudhrury et al.,46 Eiter et al.,47 and Antonini et al.51 gave IC50 values of 0.63 μM, 0.29 μM and 0.0039 μM, respectively. Thus the data revealed that these substitutions are more advantageous over the other kind of substitutions.
On similar grounds, acridine analogs as antimicrobial agents have been reported to be derivatized in a manner somewhat similar to the above mentioned derivatization, but these still lack some good support in terms of good efficacy determined by MIC values. Antimicrobial potential of acridines have been studied by making substitutions on some limited positions only.
Thus based on the results revealed by the above mentioned reports and patents, we suggest that future trends must aim at the development of acridine analogs as antimicrobial agents by making substitutions at positions where these have not been made so far. The antimicrobial discovery of acridine derivatives lacks only some good substitutions at different positions, thus, the future studies must aim at the development of the same, especially some metal complexes and hybrids of acridine, as these are expected to show good results in terms of biological activity. A mere fulfillment of this fact can lead to the discovery of some new and effective acridine analogs.
On the other hand, though a lot of work has been done on the development of anticancer agents from acridine nucleus but there are certain positions that lack any substitution so far. Thus, acridine must be derivatized at such positions (2nd, 3rd, 5th, 7th) and the derivatives must be evaluated for their anticancer potential or any other biological activity if they are expected to show such.
This review, therefore, describes acridine as one of the widely adopted N-heterocyclic system as a very efficient anticancer and antimicrobial agent. Its potential has been supported by the reports and patents described in this article. Researchers must aim at the development of some new acridine analogs with new and efficient substitutions, thus exploring this central and chief nucleus to a large extent.
ACKNOWLEDGEMENT
Declared none.
References
1. R. A. Morton, ‘Biochemistry of Quinones’, Academic Press, Inc., New York, 1965.
2. R. R. Gupta, S. K. Jain, and K. G. Ojha, Indian J. Chem., 1979, 17, 278.
3. T. Eicher and S. Hauptmann, ‘The Chemistry of Heterocycles’, Academic Press, Inc., New York, 1965.
4. W. A. Denny, Curr. Med. Chem., 2002, 9, 1655. CrossRef
5. M. Wainwright, J. Antimicrob. Chemother., 2001, 47, 1. CrossRef
6. I. Antonini, Curr. Med. Chem., 2002, 9, 1701. CrossRef
7. Z. Ma, G. Saluta, G. L. Kucera, and U. Bierbach, Bioorg. Med. Chem. Lett., 2008, 18, 3799. CrossRef
8. O. O. Fadeyi, S. T. Adamson, E. L. Myles, and C. O. Okoro, Bioorg. Med. Chem. Lett., 2008, 18, 4172. CrossRef
9. P. A. Stocks, P. G. Bray, V. E. Barton, M. Al-Helal, M. Jones, N. C. Araujo, P. Gibbons, S. A. Ward, R. H. Hughes, G. A. Biagini, J. Davies, R. Amewu, A. E. Mercer, G. Ellis, and P. M. O’Neill, Angew. Chem. Int. Ed., 2007, 46, 6278. CrossRef
10. J. H. Tan, Q. X. Zhang, Z. S. Huang, and Y. Chen, Eur. J. Med. Chem., 2006, 41, 1041. CrossRef
11. M. Kaur, S. Sharma, and P. M. S. Bedi, Chin. J. Cat., 2015, 36, 520. CrossRef
12. K. Dzierzbickam and A. M. Kolodziejczyk, J. Med. Chem., 2001, 44, 3606. CrossRef
13. J. G. Delcros, S. Tomasi, S. Carrington, B. Martin, J. Renault, I. S. Blagbrough, and P. Uriac, J. Med. Chem., 2002, 45, 5098. CrossRef
14. R. J. Harrison, J. Cuesta, G. Chessari, Read, M. A. Basra, S. K. A. P. Reszka, J. Morrell, and S. M. Gowan, J. Med. Chem., 2003, 46, 4463. CrossRef
15. S. M. Sondhi, G. Bhattacharjee, R. K. Jameel, R. Shukla, R. Raghubir, O. Lozach, and L. Meijer, CEJC, 2004, 2, 1.
16. V. A. Bacherikov, T. C. Chou, H. J. Dong, C. H. Chen, Y. W. Lin, T. J. Tsai, and T. L. Su, Bioorg. Med. Chem. Lett., 2004, 14, 4719. CrossRef
17. C. Santelli-Rouvier, J.-M. Barret, C. M. Farrell, D. Sharples, B. T. Hill, and J. Barbe, Eur. J. Med. Chem., 2004, 39, 1029. CrossRef
18. F. Delmas, A. Avellaneda, C. D. Giorgio, M. Robin, E. D. Clercq, and P. Timon-David, Eur. J. Med. Chem., 2004, 39, 685. CrossRef
19. J. R. Goodell, A. A. Madhok, H. Hiasa, and D. M. Ferguson, Bioorg. Med. Chem., 2006, 14, 5467. CrossRef
20. T.-L. Su, Y.-L. Lin, T.-C. Chou, X. Zhang, V. A. Bacherikov, C.-H. Chen, L. F. Liu, and T.-J. Tsai, J. Med. Chem., 2006, 49, 3710. CrossRef
21. C. Martins, M. Gunaratnam, J. Stuart, V. Makwana, O. Greciano, A. P. Reszka, L. R. Kelland, and S. Neidle, Bioorg. Med. Chem. Lett., 2007, 17, 2293. CrossRef
22. N. Kapuriya, K. Kapuriya, X. Zhang, T.-C. Chou, R. Kakadiya, Y.-T. Wu, T.-H. Tsai, Y.-T. Chen, T.-C. Lee, A. Shah, Y. Naliaparad, and T.-L. Su, Bioorg. Med. Chem., 2008, 16, 5413. CrossRef
23. J. R. Goodell, A. V. Ougolkov, H. Hiasa, H. Kaur, R. Remmel, D. D. Billadeau, and D. M. Ferguson, J. Med. Chem., 2008, 51, 2. CrossRef
24. L. A. Howell, A. Howman, M. A. O’Connell, A. Mueller, and M. Searcey, Bioorg. Med. Chem. Lett., 2009, 19, 5880. CrossRef
25. M. Galvez-Peralta, J. S. Hackbarth, K. S. Flatten, S. H. Kaufmann, H. Hiasa, C. Xing, and D. M. Ferguson, Bioorg. Med. Chem. Lett., 2009, 19, 4459. CrossRef
26. O. I. El-Sabbagh and H. M. Rady, Eur. J. Med. Chem., 2009, 44, 3680. CrossRef
27. D. Castillo-Gonzalez, M. A. Cabrera-Perez, M. Perez-Gonzalez, A. M. Helguera, and A. Duran-Martınez, Eur. J. Med. Chem., 2009, 44, 4826. CrossRef
28. N. K. Sathish, P. G. Kumar, V. V. S. R. Prasad, S. M. S. Kumar, and Y. C. Mayur, Med. Chem. Res., 2010, 19, 674. CrossRef
29. S. M. Sondhi, J. Singh, R. Rani, P. P. Gupta, S. K. Agrawal, and A. K. Saxena, Eur. J. Med. Chem., 2010, 45, 555. CrossRef
30. M. M. Patel, M. D. Mali, and S. K. Patel, Bioorg. Med. Chem. Lett., 2010, 20, 6324. CrossRef
31. O. I. El-Sabbagh, M. A. Shabaan, H. H. Kadry, and E. S. Al-Din, Arch. Pharm. Chem. Life Sci., 2010, 9, 519. CrossRef
32. C. Gao, F. Liu, X. Luan, C. Tan, H. Liu, Y. X. Y. Jin, and Y. Jiang, Bioorg. Med. Chem., 2010, 18, 7507. CrossRef
33. H. Paulkova, Z. Vantova, L. Hunakova, L. Cizekova, M. Carna, M. Kozurkova, D. Sabolova, P. Kristian, S. Hamulakova, and J. Imrich, Bioorg. Med. Chem. Lett., 2012, 20, 7139. CrossRef
34. M. Alvala, S. Bhatnagar, A. Ravi, V. U. Jeankumar, T. H. Manjashetty, P. Yogeeswari, and D. Sriram, Bioorg. Med. Chem. Lett., 2012, 22, 3256. CrossRef
35. R. Kalirajan,V. Kulshrestha, S. Sankar, and S. Jubie, Eur. J. Med. Chem., 2012, 56, 217. CrossRef
36. X. Lang, L. Li, Y. Chen, Q. Sun, Q. Wu, F. Liu, C. Tan, H. Liu, C. Gao, and Y. Jiang, Bioorg. Med. Chem., 2013, 21, 4170. CrossRef
37. M. F. Brana, L. Casarrubios, G. Domınguez, C. Fernandez, J. M. Perez, A. G. Quiroga, C. Navarro-Ranninger, and B. D. Pascual-Teresa, Eur. J. Med. Chem., 2002, 37, 301. CrossRef
38. I. Antonini, P. Polucci, A. Magnano, B. Gatto, M. Palumbo, E. Menta, N. Pescalli, and S. Martelli, J. Med. Chem., 2003, 46, 3109. CrossRef
39. I. Antonini, P. Polucci, A. Magnano, S. Sparapani, and S. Martelli, J. Med. Chem., 2004, 47, 5244. CrossRef
40. P. Yang, Q. Yang, and X. Qian, Tetrahedron, 2005, 61, 11895. CrossRef
41. S.-S. Wang, Y.-J. Lee, S.-C. Hsu, H.-O. Chang, W.-K. Yin, L.-S. Chang, and S.-Y. Chou, Bioorg. Med. Chem., 2007, 15, 735. CrossRef
42. Q. Liu, J. Zhang, M. Wang, D. Zhang, Q. Lu, Y. Huang, H. Lin, and X. Yu, Eur. J. Med. Chem., 2010, 45, 5302. CrossRef
43. M. G. D. R. Pitta, E. S. Souza, F. W. A. Barros, M. O. M. Filho, C. O. Pessoa, M. Z. Hernandes, M. D. C. A. D. Lima, S. L. Galdino, and I. D. R. Pitta, Med. Chem. Res., 2013, 22, 2421. CrossRef
44. T. M. Augustus, J. Anderson, S. M. Hessb, and U. Bierbach, Bioorg. Med. Chem. Lett., 2003, 13, 855. CrossRef
45. R. Guddneppanavar, J. R. Choudhury, A. R. Kheradi, B. D. Steen, G. Saluta, G. L. Kucera, C. S. Day, and U. Bierbach, J. Med. Chem., 2007, 50, 2259. CrossRef
46. J. R. Choudhury, R. Guddneppanavar, G. Saluta, G. L. Kucera, and U. Bierbach, J. Med. Chem., 2008, 51, 3069. CrossRef
47. L. C. Eiter, N. W. Hall, C. S. Day, G. Saluta, G. L. Kucera, and U. Bierbach, J. Med. Chem., 2009, 52, 6519. CrossRef
48. Z. Ma, L. Rao, and U. Bierbach, J. Med. Chem., 2009, 52, 3424. CrossRef
49. L. A. Graham, J. Suryadi, T. K. West, G. L. Kucera, and U. Bierbach, J. Med. Chem., 2012, 55, 7817. CrossRef
50. S. Ding, X. Qiao, G. L. Kucera, and U. Bierbach, J. Med. Chem., 2012, 55, 10198. CrossRef
51. I. Antonini, P. Polucci, A. Magnano, and S. Martelli, J. Med. Chem., 2001, 44, 3329. CrossRef
52. E. Delfourne, F. Darro, N. Bontemps-Subielos, C. Decaestecker, J. Bastide, A. Frydman, and R. Kiss, J. Med. Chem., 2001, 44, 3275. CrossRef
53. S. A. Gamage, J. A. Spicer, G. W. Rewcastle, J. Milton, S. Sohal, W. Dangerfield, P. Mistry, N. Vicker, P. A. Charlton, and W. A. Denny, J. Med. Chem., 2002, 45, 740. CrossRef
54. E. Delfourne, F. Darro, P. Portefaix, C. Galaup, S. Bayssade, A. Bouteille, L. L. Corre, J. Bastide, F. Collignon, B. Lesur, A. Frydman, and R. Kiss, J. Med. Chem., 2002, 45, 3765. CrossRef
55. I. Antonini, P. Polucci, A. Magnano, B. Gatto, M. Palumbo, E. Menta, N. Pescalli, and S. Martelli, J. Med. Chem., 2002, 45, 696. CrossRef
56. X. Bu, J, Chen, L. W. Deady, and W. A. Denny, Tetrahedron, 2002, 58, 175. CrossRef
57. I. Antonini, P. Polucci, A. Magnano, D. Cacciamani, M. T. Konieczny, J. Paradziej-Łukowicz, and S. Martelli, Bioorg. Med. Chem., 2003, 11, 399. CrossRef
58. a) J. C. Cookson, R. A. Heald, and M. F. G. Stevens, J. Med. Chem., 2005, 48, 7198; CrossRef b) S. Missailidis, C. Modi, V. Trapani, C. A. Laughton, and M. F. G. Stevens, Oncol. Res., 2005, 15, 95.
59. I. Sanchez, R. Reches, D. H. Caignard, P. Renard, and M. D. Pujol, Eur. J. Med. Chem., 2006, 41, 340. CrossRef
60. a) M.-K. Cheng, C. Modi, J. C. Cookson, I. Hutchinson, R. A. Heald, A. J. McCarroll, S. Missailidis, F. Tanious, W. D. Wilson, J.-L. Mergny, C. A. Laughton, and M. F. G. Stevens, J. Med. Chem., 2008, 51, 963; CrossRef b) I. Hutchinson and M. F. G. Stevens, Org. Biomol. Chem., 2007, 5, 114. CrossRef
61. S. M. Roopan and F. R. N. Khan, Med. Chem. Res., 2011, 20, 732. CrossRef
62. S.-R. Liao, C.-X. Zhou, W.-B. Wu, T.-M. Ou, J.-H. Tan, D. Li, L.-Q. Gu, and Z. S. Huang, Eur. J. Med. Chem., 2012, 53, 52. CrossRef
63. F. Alarcon, M. Demeunynck, J. Lhomme, D. Carrez, and A. Croisy, Bioorg. Med. Chem. Lett., 2001, 11, 1855. CrossRef
64. A. Kamal, O. Srinivas, P. Ramulu, G. Ramesh, and P. P. Kumar, Bioorg. Med. Chem. Lett., 2004, 14, 4107. CrossRef
65. M. Jones, A. E. Mercer, P. A. Stocks, L. J. I. L. Pensee, R. Cosstick, B. K. Park, M. E. Kennedy, I. Piantanida, S. A. Ward, J. Davies, P. G. Bray, S. L. Rawe, J. Baird, T. Charidza, O. Janneh, and P. M. O’Neill, Bioorg. Med. Chem. Lett., 2009, 19, 2033. CrossRef
66. M. Malachowska-Ugarte, G. Cholewinski, K. Dzierzbicka, and P. Trzonkowski, Eur. J. Med. Chem., 2012, 54, 197. CrossRef
67. F. Charmantray, M. Demeunynck, D. Carrez, A. Croisy, A. Lansiaux, C. Bailly, and P. Colson, J. Med. Chem., 2003, 46, 967. CrossRef
68. C. Asche, P. Dumy, D. Carrez, A. Croisy, and M. Demeunynck, Bioorg. Med. Chem. Lett., 2006, 16, 1990. CrossRef
69. C. D. Giorgio, K. Shimi, G. Boyer, F. Delmas, and J.-P. Galy, Eur. J. Med. Chem., 2007, 42, 1277. CrossRef
70. Y. Benchabane, C. D. Giorgio, G. Boyer, A.-S. Sabatier, D. Allegro, V. Peyrot, and M. D. Meo, Eur. J. Med. Chem., 2009, 44, 2459. CrossRef
71. L. Janovec, M. Kozurkova, D. Sabolova, J. Ungvarsky, H. Paulikova, J. Plsikova, Z. Vantova, and J. Imrich, Bioorg. Med. Chem., 2011, 19, 1790. CrossRef
72. E. A. Ankers, B. J. Evison, D. R. Phillips, R. T. C. Brownlee, and S. M. Cutts, Bioorg. Med. Chem. Lett., 2014, 24, 5710. CrossRef
73. G. J. Atwell, B. C. Baguley, W. A. Rewcastle, and G. W. Denny, US4603125A, 1986.
74. K. A. Watanabe and K. Takahashi, US5229395A, 1993.
75. B. C. Baguley, G. J. Atwell, W. A. Denny, G. J. Finlay, and G. W. Rewcastle, WO9324096A2, 1993.
76. W. A. Denny, S. Gamage, J. A. Spicer, B. C. Baguley, and G. J. Finlay, EP0934278B1, 1998.
77. G. W. Gribble, G. D. Jaycox, and M. Mosher, WO1998057956A1, 1998.
78. I. Antonini, S. Martelli, and P. Polucci, WO9906372A1, 1998.
79. Y. B. Han, H. K. Kyung, C. W. Kim, E. T. Ahn, K. Hong, H. S. Choi, S. G. Kim, B. I. Yoo, and H. K. Keon, US5891864A, 1999.
80. M. D. Snodin and E. P. Clarkson, WO2006095139A2, 2006.
81. S. Neidle, R. H. Harrison, L. R. Kelland, S. M. Gowan, and M. R. Read, WO0208193A2, 2002.
82. S. Neidle, R. H. Harrison, L. R. Kelland, S. M. Gowan, M. R. Read, S. Waldon, M. A. Read, and A. Reszka, US7205311B2, 2007.
83. I. D. R. Pitta, L. G. Suely, A. D. Lima, and M. D. Carmo, WO2007109871A2, 2007.
84. T.-L. Su and T.-C. Chou, US20080176889A1, 2008.
85. U. Bierbach and Winston-Salem, US8906896B2, 2014.
86. U. Bierbach and Winston-Salem, CA2741683A1, 2010.
87. U. Bierbach and Winston-Salem, WO2010048499A1, 2010.
88. U. Bierbach and S. Ding, US2014193334A1, 2014.
89. M. D. C. Carvalho, G. S. Palva, and I. A. D. Souza, WO2013091052A1, 2013.
90. L. Ngadi, A. M. Galy, J. P. Galy, J. Barbe, A. Cremieux, J. Chevalier, and D. Sharples, Eur. J. Med. Chem., 1990, 25, 67. CrossRef
91. J. Cremieux, D. Chevalier, H. Sharples, A. M. Berny, P. Galy, J. P. Brouant, and J. Barbe, Res. Microbiol., 1995, 146, 73. CrossRef
92. K. Nesmerak, M. Pospisek, B. Zikanova, I. Nemec, J. Barbe, and J. Gabriel, Folia Microbiol., 2001, 47, 118. CrossRef
93. A. Stankiewicz-Drogon, L. G. Palchykovska, V. G. Kostina, I. V. Alexeeva, A. D. Shved, and A. M. Boguszewska-Chachulska, Bioorg. Med. Chem., 2008, 16, 8846. CrossRef
94. T. E. S. Ali and A. M. El‐Kazak, Eur. J. Chem., 2010, 1, 6. CrossRef
95. N. P. Singh, R. Kumar, D. N. Prasad, S. Sharma, and O. Silakari, Int. J. Biol. Chem., 2011, 5, 193. CrossRef
96. R. Kalirajan, V. Muralidharan, S. Jubie, and S. Sankar, Int. J. Health Allied Sci., 2013, 2, 81. CrossRef
97. L. R. Daghigh, M. Pordel, and A. Davoodnia, J. Chem. Res., 2014, 38, 202. CrossRef
98. A. R. Benoit, C. Schiaffo, C. E. Salomon, J. R. Goodell, H. Hiasa, and D. M. Ferguson, Bioorg. Med. Chem. Lett., 2014, 24, 3014. CrossRef
99. L. Ngadi, N. G. Bisri, A. Mahamoud, A. M. Galy, J. P. Galy, J. C. Soyfer, J. Barbe, and M. Placidi, Arzneim. Forsch., 1993, 43, 480.
100. M. Rahimizadeh, M. Pordel, M. Bakavoli, Z. Bakhtiarpoor, and A. Orafaie, Monatsh. Chem., 2009, 140, 633. CrossRef
101. N. Bontemps, D. Bry, S. Lopez-Legentil, A. Simon-Levert, C. Long, and B. Banaigs, J. Nat. Prod., 2010, 73, 1044. CrossRef
102. K. Prabakaran, E. Yamuna, and K. J. R. Prasad, Ind. J. Chem., 2011, 50, 906.
103. S. G. Konda and B. S. Dawane, Int. J. Pharm. Sci. Rev. Res., 2012, 15, 121.
104. D. G. Carole, D. M. Michel, C. Julien, D. Florence, N. Anna, J. Severine, D. Gerard, T.-D. Pierre, and G. Jean-Pierre, Bioorg. Med. Chem., 2005, 13, 5560. CrossRef
105. E. S. H. E. Ashry, L. F. Awad, E. L. I. Ibrahim, and O. K. Bdeewy, ARKIVOC, 2006, ii, 178.
106. H. Seneca and F. Lee, US31222553A, 1964.
107. R. Cohn, IL33296A, 1973.
108. Eitan, Pearl, Latzer, and Zedek, IL36136A, 1974.
109. J. W. Schulenberg, US4150134A, 1979.
110. A. Kovacs, A. Liptak, P. Nanasi, J. Janossy, I. Csernas, J. Erdei, I. Kaszab, K. Polya, and A. Neszmelyi, US4462993, 1984.
111. D. B. Capps, EP0138302A1, 1985.
112. A. Denys, G. Jerzy, and A. Jan, WO9830544, 1998.
113. M. K. Riscoe, R. Winter, J. X. Kelly, D. J. Hinrichs, and M. J. Smilkstein, US20100069428A1, 2010.
114. J. L. Edmond, A. Parhi, and S. Pilch, WO201116610A2, 2011.
115. a) T. Eicher and S. Hauptmann, ‘The Chemistry of Heterocycles. Structure, Reactions, Syntheses and Applications’, George Thieme Verlag, Stuttgart, New York, 1995; b) D. J. Abraham, ‘Burgers Medicinal Chemistry and Drug Discovery’, John Wiley and Sons, New York, 2007; c) F. G. Mann and B. C. Saunders, ‘Practical Organic Chemistry’, Orient Longman Private Ltd., New Delhi, 2003.
116. a) F. C. Bruce, N. S. Ralph, and J. A. Graham, J. Med. Chem., 1974, 17, 923; CrossRef b) A. Bernthsen, Ann., 1884, 1, 224.
117. R. M. Acheson, ‘Introduction to the Chemistry of Heterocyclic Compounds’, Wiley Interscience, New York, 2008.
118. R. Kumar, M. Kaur, and M. Kumari, ‘Acridine: A Versatile Heterocyclic Nucleus’, Acta Pol. Pharm. Drug Res., 2012, 69, 3.