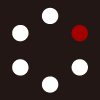
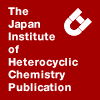
HETEROCYCLES
An International Journal for Reviews and Communications in Heterocyclic ChemistryWeb Edition ISSN: 1881-0942
Published online by The Japan Institute of Heterocyclic Chemistry
e-Journal
Full Text HTML
Received, 24th October, 2015, Accepted, 25th November, 2015, Published online, 8th December, 2015.
DOI: 10.3987/COM-15-13349
■ An Efficient Synthesis of 2-Selenouridine and Its Phosphoramidite Precursor
Masakazu Kogami, Darrell R. Davis, and Mamoru Koketsu*
Department of Chemistry and Biomolecular Science, Faculty of Engineering, Gifu University , 1-1 Yanagido, Gifu, Gifu 501-1193, Japan
Abstract
We describe an efficient and scalable synthetic route to the natural occurring 2-selenouridine (Se2U) and its suitably protected derivative for phosphoramidite synthesis. From known fully TBDMS-protected thiouridine, the corresponding selenouridine was synthesized via the reaction of methylthiouridine with NaHSe, which was then completely deprotected without affecting the selenocarbonyl moiety to deliver Se2U. Next, Se2U was converted into the 2′-O-TBDMS-5′-O-DMT derivative in 81% yield over four steps including di-tert-butylsilylene (DTBS) introduction at O3′ and O5′, O2′-silylation, selective cleavage of DTBS, and DMT introduction. This synthetic route enabled gram-scale preparation of both Se2U and its phosphoramidite precursor in excellent yields. Furthermore, the predicted C3′-endo conformational preference of Se2U was experimentally confirmed by NMR spectroscopy.INTRODUCTION
Selenium is an essential trace element for humans and animals and used in a wide variety of biological process.1 It is incorporated into selenoproteins in the form of selenocysteine (Sec) for the reduction of antioxidant enzymes such as glutathione peroxidases.2 Furthermore, selenium modified nucleosides have proven to be useful in biochemistry, molecular biology, gene therapy, and drug discovery.3 The covalent radius of sulfur (1.05 A˚) is larger than that of oxygen (0.66 A˚) and although sulfur is less electronegative, it is more polarizable.4 The replacement of oxygen at the nucleobase C-2 position with sulfur stabilizes the sugar C3′-endo conformation primarily through steric repulsion between the 2-thiocarbonyl group and sugar 2′-hydroxy group.5 Moreover, 2-thiouridine (S2U) selectively destabilizes the S2U/G wobble-pair geometry relative to an unmodified U primarily due to the weaker hydrogen bonding ability of sulfur relative to oxygen.6 Therefore, the naturally occurring selenium (1.20 A˚)4 modification of nucleobase C-2 position is expected to be even more efficient than sulfur modification in mediating these effects. Recently, Huang et al. reported that selenium modification at the nucleobase C-2 position confers greater base pairing specificity compared to unmodified uridine.7
Thiouridine and selenouridine nucleoside modifications may be therapeutically relevant since in human immunodeficiency virus type-1 (HIV-1) a key step in the viral life cycle requires base pairing of the reverse transcriptase primer, human tRNALys3, to the HIV-1 genomic RNA.8 This interaction of viral RNA and human tRNALys3 initiates viral reverse transcription and key interactions between sulfur-modified nucleosides in the tRNA anticodon domain and a conserved region of the HIV genome are necessary for efficient viral replication. Functional study of nucleobase modification in the initiation complex of HIV-1 reverse transcription is important since targeting this step could be a target for new drugs.9 Modification of nucleosides with S2U in tRNALys3 stabilized the RNA-RNA interaction,10 that is used by the viral reverse transcriptase as a primer.11 Oligonucleotides containing selenium modification could also mimic the interaction between the native tRNA and the HIV primer binding site, and inhibit viral replication more effectively than either sulfur or unmodified oligonucleotide therapeutics.
The structure of 2-selenouridine (Se2U) and its derivatives discovered from tRNAGlu, tRNAGln, and tRNALys in Escherichia coli or in Methanococcus vannielii are shown in Figure 1.12 The YbbB enzyme
necessary for forming Se2U from U has been shown to present in all kingdoms of life, underscoring the importance of this ubiquitous, yet minimally studied nucleoside modification.13
Despite the biological importance, understanding the functional role of selenium modified nucleosides is limited by the availability of these nucleosides.14 Few reports are available in the literature for the synthesis of 2-selenouridine due to difficulties involved in their preparation. Thus, it is useful to have a scalable route to prepare these nucleosides for both structural and biological study.
To the best of our knowledge, Se2U has been synthesized by five research groups. The first method15a required difficult to obtain 2-selenouracil16 that was synthesized from expensive and unstable N,N-unsubstituted selenourea and sodium ethylformyl acetate. Furthermore, glycosylation of silylated 2-selenouracil with glycosyl donors in the presence of tin(IV) chloride provided only a 30% yield. The second synthesis of Se2U was accomplished by ipso-substitution of isocytidine with gaseous H2Se.15b This route seems to be the shortest. However, this method requires the use of a difficult to obtain starting material isocytidine that was synthesized from 2-thiouracil in 5 steps,17 and then required highly toxic gaseous H2Se, and long reaction times (3 days). In these initial reports, the full characterization and experimental details for preparing Se2U was not provided. Recently, the synthesis of Se2U has been reported independently by Huang′s group,15c Moderna Therapeutics,15d and Nawrot′s group.15e Huang′s method provided the critically useful Se2U-phosphoramidite, which could be synthesized from 2-thiouracil in 8 steps. However, this method gave a nearly 1:1 mixture of undesired but separable 2′- and 3′-O-silyl ether derivatives prior to selenium protection as the Se-cyanoethyl, thus the desired 2′-O-silyl ether derivative was obtained in 41% yield. The Moderna Therapeutics method provided Se2U from 2-thiouracil in 7 steps. Therefore, their route seems to need improvement for synthetic production on a large scale. Herein, we describe an efficient and scalable synthetic route that allows gram scale synthesis, avoidance of tedious isomer purification through regioselective 2′-O-silylation, and finally the mild deprotection of a triol protecting group on Se2U.
RESULTS AND DISCUSSION
In our route outlined in Scheme 1, we focused on increasing efficiency based on diverse view point.18 First, 2′,3′,5′-O-tris(tert-butyldimethylsilyl) (TBDMS)-2-thiouridine (2) was obtained from commercially available and inexpensive uridine (1) (ca. $3.3/gram, Tokyo Chemical Industry Co., Ltd., October 2015) on the 10.4 g scale in 55% overall yield according to the literature method.19 Sekine′s method provides several advantages over the glycosylation method, involving avoidance of the moisture-sensitive reaction, inseparable emulsion during the extraction due to insoluble tin(IV) chloride and easy access to the highly-soluble per-silylated intermediate.
Next, we carried out the S-alkylation of 2 with methyl iodide in the presence of an aqueous sodium hydroxide in THF to afford the corresponding 2′,3′,5′-O-TBDMS-2-methylthiouridine (3) in excellent yield. This biphasic condition produced almost a single product, thereby eliminating the requirement for silica gel chromatography. The resulting 3 was subsequently treated with NaHSe, which was generated by reduction of selenium with sodium borohydride in EtOH at 60 °C, to afford 6.5 g of 2′,3′,5′-O-TBDMS-2-selenouridine (4) in 90% yield from 2.
After extensive optimization, mild desilylation of 4 was accomplished by using aqueous trifluoroacetic acid (TFA) to yield 2.3 g (94% yield) of 5. When tetra-n-butylammonium fluoride (TBAF) was used as a deprotection reagent, the yield dropped dramatically to 31%.15d The treatment with TBAF caused deselenation to give the mixture of uridine and selenium as byproducts, which required a multi-step, tedious silica gel column chromatography procedure to separate these products from 5. In contrast to the previously reported synthesis, the mild deprotection using aqueous TFA offers the following advantages: (i) complete absence of depyrimidination and deselenation from acidic hydrolysis of the nucleobase; (ii) a facile purification process including only simple precipitation of 5 with diethyl ether. The structure of 5 was confirmed by 1H, 13C NMR and 77Se NMR spectra compared to the previous report.15e The production of a newly formed 2-selenocarbonyl group of 5 provided a 77Se NMR peak at 353.8 ppm.
With a scalable (>2 g) route to Se2U secured, our attention turned to the Se2U-phosphoramidite precursor. Widely-used solid-phase RNA chemical synthesis offers advantages for the preparation of oligoribonucleotides, however, facile and relatively inexpensive access to the phosphoramidite is required. A major drawback in the previously reported method is non-regio-selective protection for the secondary hydroxyls of Se2U with TBDMS groups. Due to this non-selectivity, there is generally an arduous purification step to separate the desired 2′-O-silyl derivative from its 3′ regio-isomer.
To solve this problem, we tried 5′-O-dimethoxytritylation, followed by selective 2′-O-silylation using silver nitrate.20 However, the desired 2′-O-silyl compound was not obtained due to decomposition from oxidization of the selenocarbonyl group. Next, we employed the di-tert-butylsilylene (DTBS) group21 for simultaneous protection of 3′- and 5′-hydroxyl functions of 5. The reaction of 5 (1.2 g) with 1.1 equiv. of di-tert-butylsilyl bis(trifluoromethanesulfonate) (DTBS(OTf)2) in DMF at 0 °C proceeded smoothly to afford the 3′,5′-O-protected intermediate, which was subsequently silylated at O-2′ in one pot, producing 2.0 g of isolated product (93% yield from 5). Then, selective removal of DTBS group using hydrogen fluoride–pyridine in dichloromethane at 0 °C furnished the 2′-O-TBDMS Se2U, which was then subjected to next reaction without chromatographic purification. Reaction with 4,4′-dimethoxytrityl chloride (DMT-Cl) in pyridine gave 2.3 g of compound 7 in 87% yield from 6, which can be converted to the Se2U-phosphoramidite 8 in 2 steps.15c As a result, we succeeded in the synthesis of phosphoramidite precursor 7 in 38% over 11 steps, from the inexpensive, commercially available uridine 1.
In order to investigate the structural characteristics of the 2-selenocarbonyl group of Se2U, the 1H NMR spectra of 5 was acquired. As mentioned above, sulfur modification in S2U has been shown to generally stabilize the sugar C3′-endo conformation because of steric repulsion between 2-thiocarbonyl group and 2′-hydroxyl group.
Therefore, selenium with a covalent radius of 1.20 A˚ when replacing oxygen at the nucleobase C-2 position is expected to stabilize the sugar conformation even more. As shown in Table 1, the % [C3′-endo] value of the Se2U 5 was calculated to be 80%, whereas for U and S2U it were 53% and 71%,22 respectively. This result indicated that 2-selenium modification does indeed stabilize the sugar C3′-endo conformation at the nucleoside level.
In conclusion, each of the synthetic steps in our optimized procedure has been successfully conducted on a gram scale, attesting to the scalability and efficacy of this route. In addition, we have applied this methodology to the synthesis of the Se2U phosphoramidite precursor, demonstrating the feasibility of this route for obtaining Se2U containing RNA oligonucleotides. The presented synthesis of Se2U will contribute to further functional studies on Se2U regarding to the initiation complex of HIV-1 reverse transcription. Se2U will also be useful as a tractable key intermediate that is applicable to the synthesis of other natural occurring selenium-containing pyrimidine nucleosides in tRNAs. The synthesis of these nucleosides is ongoing in our laboratory.
EXPERIMENTAL
Commercially available reagents were used without further purification. All reactions were performed in a dry solvent under an argon atmosphere. Transfer of air- and moisture-sensitive reagents was performed via cannula under a positive pressure of argon atmosphere. DMF was degassed by argon bubbling prior to use. Analytical thin-layer chromatography (TLC) analysis was performed on Merck TLC (silica gel 60F254 on glass plate). The developed chromatography was analyzed by UV lamp (254 nm) or by spraying 10% H2SO4 solution in EtOH, followed by heating. Silica gel 60N (spherical, neutral) manufactured by Kanto Chemical Co., Inc. was used for flash column chromatography. NMR spectra were recorded on JEOL JNM-ECA600 (1H NMR: 600 MHz, 13C NMR: 150 MHz, 77Se NMR: 113 MHz) spectrometer. Chemical shifts of 1H NMR are reported in δ values referenced to CHCl3 (δ 7.26 ppm) or D2O (δ 4.79 ppm) as an internal standard and the following abbreviation were used as follows: s: singlet, d: doublet, t: triplet, m: multiplet. Chemical shifts of 13C NMR are reported in δ values referenced to CHCl3 (δ 77.1 ppm) as an internal standard. The 77Se NMR chemical shifts are reported in ppm (δ) relative to the external standard. High-resolution mass spectra (HRMS) was measured with a Waters UPLC system (Aquity UPLC XevoQTof). Melting points were measured by a Yanagimoto micromelting point apparatus. IR spectra were measured on JASCO FT/IR-410 Fourier Transform Infrared Spectrometer in CHCl3 or MeOH. Optical rotations were measured with Union PM-201 Automatic Digital Polarimeter.
2′,3′,5′-O-Tris(tert-butyldimethylsilyl)-2-methylthiouridine (3)
To a solution of 2 (6.70 g, 11.12 mmol) in a mixture of THF (20 mL) and 10% NaOH aq. (20 mL) was added methyl iodide (0.83 mL, 13.34 mmol) at rt. The mixture was stirred for 4 h at rt (TLC monitoring; EtOAc:n-hexane = 1:2). The reaction mixture was extracted with CH2Cl2 (80 mL), and the organic layer was washed with water (40 mL), dried over Na2SO4, and concentrated in vacuo to afford 3 as a white foam. Compound 3 was then used in the next step without further purification.
[α]D24 -60.5 (c 0.2, CHCl3).
IR (film): 2929, 2897, 2858, 1658, 1476, 1445, 1349, 1257, 1221, 1157, 1108, 1071, 1036, 1004, 963, 938, 891, 833, 778 cm-1.
1H NMR (600 MHz, CDCl3): δ = 7.92 (d, 1H, J = 7.6 Hz, H-6), 6.05 (d, 1H, J = 7.6 Hz, H-5), 5.92 (d, 1H, J = 6.9 Hz, H-1′), 4.13 (dd, 1H, J = 6.8, 4.8 Hz, H-2′), 4.05-4.04 (m, 2H, H-3′, H-4′), 3.87 (dd, 1H, J = 11.7, 2.8 Hz, H-5′b), 3.72 (dd, 1H, J = 11.7, 1.4 Hz, H-5′a), 2.56 (s, 3H, SMe), 0.93 (s, 9H, tBu), 0.92 (s, 9H, tBu), 0.84 (s, 9H, tBu), 0.12 (s, 6H, Si(CH3)2), 0.10 (s, 3H, Si(CH3)2), 0.07 (s, 3H, Si(CH3)2), -0.02 (s, 3H, Si(CH3)2), -0.16 (s, 3H, Si(CH3)2).
13C NMR (150 MHz, CDCl3): δ = 168.6, 163.7, 138.5, 109.9, 90.6, 87.2, 73.2, 63.4, 26.1, 25.91, 25.88, 18.5, 18.2, 18.0, 14.8, -4.4, -4.9, -5.41, -5.45.
HRMS (ESI): calcd for C28H56N2O5SSi3Na [M+Na]+: 639.3116; found: 639.3115.
2′,3′,5′-O-Tris(tert-butyldimethylsilyl)-2-selenouridine (4)
Sodium borohydride (1.77 g, 46.70 mmol) was added to a suspension of Se (3.06 g, 38.92 mmol) in degassed EtOH (30 mL) at 0 °C under an argon atmosphere, and the mixture was stirred for 30 min. After this time, compound 3 was added at 0 °C. The resulting solution was stirred for 4 h at 60 °C (TLC monitoring; EtOAc:n-hexane = 1:2), then cooled to rt, the reaction mixture was extracted with EtOAc (100 mL), and the organic layer was washed twice with water (40 mL) and brine (40 mL), dried over Na2SO4, and then solvent removed by evaporation. The residue was purified by column chromatography on silica gel (EtOAc:n-hexane = 1:20 to 1:5) to afford 4 as a yellow foam; yield: 6.52 g (90%, 2 steps); [α]D24 +20.0 (c 0.2, CHCl3).
IR (film): 3087, 2929, 2858, 1686, 1618, 1471, 1441, 1389, 1362, 1256, 1216, 1166, 1134, 1109, 1074, 1053, 1037, 996, 964, 939, 836 cm-1.
1H NMR (600 MHz, CDCl3): δ = 10.84 (br s, 1H, NH), 8.38 (d, 1H, J = 7.6 Hz, H-6), 6.56 (d, 1H, J = 2.8 Hz, H-1′), 6.08 (d, 1H, J = 8.2 Hz, H-5), 4.27-4.26 (m, 1H, H-2′), 4.15 (d, 1H, J = 6.2 Hz, H-4′), 4.06-4.02 (m, 2H, H-3′, H-5′b), 3.79 (d, 1H, J = 12.4 Hz, H-5′a), 0.94 (s, 9H, tBu), 0.904 (s, 9H, tBu), 0.897 (s, 9H, tBu), 0.17 (s, 3H, Si(CH3)2), 0.13 (s, 3H, Si(CH3)2), 0.12 (s, 3H, Si(CH3)2), 0.11 (s, 3H, Si(CH3)2), 0.09 (s, 3H, Si(CH3)2), 0.07 (s, 3H, Si(CH3)2).
13C NMR (150 MHz, CDCl3): δ = 176.8, 159.2, 141.4, 107.8, 95.7, 84.5, 70.5, 61.4, 26.18, 26.16, 26.08, 18.7, 18.34, 18.31, -3.5, -3.9, -4.4, -4.7, -5.1, -5.5.
77Se NMR (113 MHz, CDCl3): δ = 423.4.
HRMS (ESI): calcd for C27H54N2O5SeSi3Na [M+Na]+: 673.2403; found: 673.2405.
2-Selenouridine (5)
Compound 4 (5.27 g, 8.12 mmol) was added to a mixture of TFA (21 mL) and water (14 mL). The resulting solution was stirred for 6 h at rt (TLC monitoring; CHCl3:acetone = 1:1). The reaction mixture was co-evaporated with toluene in vacuo, the resulting precipitate was filtered, then washed with Et2O (60 mL) to afford 5 as a white solid; yield: 2.35 g (94%); Mp 186.5-188.5 °C (H2O); [α]D24 -20.0 (c 0.1, DMSO).
IR (film): 2917, 2834, 2605, 1778, 1712, 1622, 1472, 1433, 1396, 1257, 1030, 840, 745 cm-1.
1H NMR (600 MHz, D2O): δ = 8.11 (d, 1H, J = 8.3 Hz, H-6), 6.61 (d, 1H, J = 2.0 Hz, H-1′), 6.21 (d, 1H, J = 8.3 Hz, H-5), 4.35 (d, 1H, J = 2.0 Hz, H-2′), 4.12 (m, 2H, H-3′, H-4′), 3.94 (d, 1H, J = 11.7 Hz, H-5′b), 3.79 (d, 1H, J = 13.1 Hz, H-5′a).
13C NMR (150 MHz, D2O): δ = 175.7, 161.7, 141.6, 108.1, 95.9, 84.0, 74.9, 68.3, 59.7.
77Se NMR (113 MHz, D2O): δ = 353.8.
HRMS (ESI): calcd for C9H12N2O5SeNa [M+Na]+: 330.9809; found: 330.9820.
2′-O-tert-Butyldimethylsilyl-3′,5′-O-(di-tert-butylsilanediyl)-2-selenouridine (6)
Compound 5 (1.20 g, 3.92 mmol) was suspended in DMF (10 mL) and di-tert-butylsilyl bis(trifluoromethanesulfonate) (1.40 mL, 4.31 mmol) was added dropwise over 5 min while the solution was stirred at 0 °C. The resulting solution was stirred for 40 min at 0 °C (TLC monitoring; EtOAc:n-hexane = 1:1) and then imidazole (1.33 g, 19.6 mmol) added to the solution at 0 °C. The mixture was stirred for 5 min at 0 °C and further stirred for 25 min at rt. tert-Butyldimethylchlorosilane (0.89 g, 5.88 mmol) was added to the solution at rt and the reaction allowed to proceed at 60 °C for 2 h (TLC monitoring; EtOAc:n-hexane = 1:1). The reaction mixture was extracted with EtOAc (60 mL), and the organic layer was washed with sat. NaHCO3 aq. (30 mL) and brine (30 mL), dried over Na2SO4, and then evaporated. The reaction mixture was co-evaporated with toluene under reduced pressure. The resulting residue was purified by column chromatography on silica gel (EtOAc:n-hexane = 1:15 to 1:4) to afford 6 as a yellow foam; yield: 2.04 g (93%); [α]D24 +22.0 (c 0.2, CHCl3).
IR (film): 3098, 2934, 2895, 2860, 1689, 1625, 1471, 1438, 1387, 1260, 1167, 1126, 1064, 896, 828, 757 cm-1.
1H NMR (600 MHz, CDCl3): δ = 7.33 (d, 1H, J = 8.3 Hz, H-6), 6.66 (s, 1H, H-1′), 6.14 (d, 1H, J = 8.2 Hz, H-5), 4.55 (dd, 1H, J = 9.6, 4.8 Hz, H-5′b), 4.36 (d, 1H, J = 4.8 Hz, H-2′), 4.25 (ddd, 1H, J = 9.6, 9.6, 5.5 Hz, H-4′), 4.03-4.00 (m, 1H, H-5′a), 3.80 (dd, 1H, J = 9.6, 4.8 Hz, H-3′), 1.05 (s, 9H, tBu), 1.03 (s, 9H, tBu), 0.95 (s, 9H, tBu), 0.25 (s, 3H, Si(CH3)2), 0.16 (s, 3H, Si(CH3)2).
13C NMR (150 MHz, CDCl3): δ = 176.8, 158.3, 139.4, 108.4, 99.2, 76.4, 76.0, 75.2, 67.7, 27.6, 27.1, 26.1, 23.0, 20.5, 18.4, -3.2, -4.2.
77Se NMR (113 MHz, CDCl3): δ = 448.4.
HRMS (ESI): calcd for C23H42N2O5SeSi2Na [M+Na]+: 585.1695; found: 585.1707.
5′-O-(4,4′-Dimetoxytrityl)-2′-O-tert-butyldimethylsilyl-2-selenouridine (7)
Hydrogen fluoride–pyridine (0.54 mL, 21.70 mmol) was carefully diluted with pyridine (2.34 mL, 28.96 mmol) at 0 °C. The resulting solution was added slowly to a stirred suspension of 6 (2.03 g, 3.62 mmol) in CH2Cl2 (20 mL) at 0 °C and the reaction was allowed to proceed for 3 h at 0 °C (TLC monitoring; EtOAc:n-hexane = 1:1). The reaction mixture was diluted with CH2Cl2 (20 mL), and washed with water (20 mL) and sat. NaHCO3 aq. (20 mL). The organic layer was dried over Na2SO4 and concentrated in vacuo to afford 2′-O-tert-butyldimethylsilyl-2-selenouridine. To a stirred solution of 2′-O-tert-butyldimethylsilyl-2-selenouridine in pyridine (10 mL) at 0 °C was added 4,4′-dimethoxytrityl chloride (2.45 g, 7.24 mmol). The reaction mixture was stirred for 2 h at rt (TLC monitoring; EtOAc:n-hexane = 1:1), quenched with anhydrous EtOH (2.0 mL) and concentrated under reduced pressure. The reaction mixture was extracted with CH2Cl2 (40 mL) and the organic layer was washed with sat. NaHCO3 aq. (20 mL) and brine (20 mL), then dried over Na2SO4, and concentrated under reduced pressure. The residue was purified by column chromatography on silica gel (EtOAc:n-hexane = 1:10 to 1:2) to afford 7 as a yellow foam; 2.29 g (87%, 2 steps); [α]D24 +4.50 (c 0.2 , CHCl3).
IR (film): 3087, 3016, 2952, 2930, 2857, 2838, 1691, 1608, 1582, 1509, 1465, 1442, 1254, 1177, 1122, 1036, 836, 756 cm-1.
1H NMR (600 MHz, CDCl3): δ = 11.18 (br s, 1H, NH), 8.25 (d, 1H, J = 7.6 Hz, H-6), 7.35-7.23 (m, 9H, Ph), 6.87 (d, 1H, J = 3.5 Hz, H-1′), 6.85-6.83 (m, 4H, Ph), 5.51 (d, 1H, J = 8.3 Hz, H-5), 4.50-4.49 (m, 1H, H-2′), 4.43-4.41 (m, 1H, H-3′), 4.20-4.19 (m, 1H, H-4′), 3.79 (s, 6H, OMe), 3.56-3.51 (m, 2H, H-5′a, H-5′b), 2.67 (d, 1H, J = 5.5 Hz, OH), 0.94 (s, 9H, tBu), 0.24 (s, 3H, Si(CH3)2), 0.17 (s, 3H, Si(CH3)2).
13C NMR (150 MHz, CDCl3): δ = 177.3, 159.1, 158.9, 158.8, 144.2, 141.4, 134.9, 134.7, 130.3, 130.1, 128.2, 128.1, 127.4, 113.4, 108.2, 95.5, 87.5, 84.2, 70.9, 62.3, 55.4, 25.9, 18.3, -3.9, -4.8.
77Se NMR (113 MHz, CDCl3): δ = 413.4.
HRMS (ESI): calcd for C36H44N2O7SeSiNa [M+Na]+: 747.1981; found: 747.1996.
ACKNOWLEDGEMENTS
This work was supported by a Grant-in-Aid for Scientific Research from the Ministry of Education, Culture, Sports, Science and Technology of Japan (No. 15K07856) to which we are grateful.
References
1. K. M. Brown and J. R. Arthur, Public Health Nutr., 2001, 4, 593; CrossRef C. D. Thomson, Eur. J. Clin. Nutr., 2004, 58, 391; CrossRef L. R. Ferguson, N. Karunasinghe, S. Zhu, and A. H. Wang, Mutat. Res., 2012, 733, 100. CrossRef
2. J. T. Rotruck, A. L. Pope, H. E. Ganther, A. B. Swanson, D. G. Hafeman, and W. G. Hoekstra, Science, 1973, 179, 588; CrossRef J. T. Deagen, J. A. Butler, M. A. Beilstein, and P. D. Whanger, J. Nutr., 1987, 117, 91; L. Johansson, G. Gafvelin, and E. S. Arnér, Biochim. Biophys. Acta, 2005, 1726, 1.
3. For a comprehensive review on modified nucleosides, see: W. R. Wagner, Nature, 1994, 372, 333; CrossRef J. B. Opalinska and A. M. Gewirtz, Nat. Rev. Drug Disc., 2002, 1, 503; CrossRef N. S. Que-Gewirth and B. A. Sullenger, Gene Ther., 2007, 14, 283; CrossRef J. A. Broderick and P. D. Zamore, Gene Ther., 2011, 18, 1104; CrossRef L. P. Jordheim, D. Durantel, F. Zoulim, and C. Dumontet, Nat. Rev. Drug Disc., 2013, 12, 447; CrossRef D. de Souza, D. O. Mariano, F. Nedel, E. Schultze, V. F. Campos, F. Seixas, R. S. da Silva, T. S. Munchen, V. Ilha, L. Dornelles, A. L. Braga, J. B. Rocha, T. Collares, and O. E. Rodrigues, J. Med. Chem., 2015, 58, 3329. CrossRef
4. B. Cordero, V. Gómez, A. E. Platero-Prats, M. Revésm, J. Echeverría, E. Cremades, F. Barragán, and S. Alvarez, Dalton Trans., 2008, 7, 2832. CrossRef
5. Y. Yamamoto, S. Yokoyama, T. Miyazawa, K. Watanabe, and S. Higuchi, FEBS Lett., 1983, 157, 95; CrossRef S. Yokoyama, T. Watanabe, K. Murao, H. Ishikura, Z. Yamaizumi, S. Nishimura, and T. Miyazawa, Proc. Natl. Acad. Sci. USA, 1985, 82, 4905; CrossRef H. Sierzputowska-Gracz, E. Sochacka, A. Malkiewicz, K. Kuo, C. W. Gehrke, and P. F. Agris, J. Am. Chem. Soc., 1987, 109, 7171. CrossRef
6. K. Shohda, I. Okamoto, T. Wada, K. Seio, and M. Sekine, Bioorg. Med. Chem. Lett., 2000, 10, 1795; CrossRef E. Sochacka, R. H. Szczepanowski, M. Cypryk, M. Sobczak, M. Janicka, K. Kraszewska, P. Bartos, A. Chwialkowska, and B. Nawrot, Nucleic Acids Res., 2015, 43, 2499. CrossRef
7. A. E. Hassan, J. Sheng, W. Zhang, and Z. Huang, J. Am. Chem. Soc., 2010, 132, 2120. CrossRef
8. C. Isel, R. Marquet, G. Keith, C. Ehresmann, and B. Ehresmann, J. Biol. Chem., 1993, 268, 25269; C. Isel, E. Westhof, C. Massire, S. F. Le Grice, B. Ehresmann, C. Ehresmann, and R. Marquet, EMBO J., 1999, 18, 1038. CrossRef
9. T. E. Abbink and B. Berkhout, Virus Res., 2008, 134, 4; CrossRef C. Isel, C. Ehresmann, and R. Marquet, Viruses, 2010, 2, 213; CrossRef S. Liu, B. T. Harada, J. T. Miller, S. F. Le Grice, and X. Zhuang, Nat. Struct. Mol. Biol., 2010, 17, 1453. CrossRef
10. R. K. Kumar and D. R. Davis, Nucleic Acids Res., 1997, 25, 1272. CrossRef
11. M. Sundaram, P. F. Crain, and D. R. Davis, J. Org. Chem., 2000, 65, 5609; CrossRef M. Sundaram, P. C. Durant, and D. R. Davis, Biochemistry, 2000, 39, 12575; CrossRef A. C. Bajji and D. R. Davis, Org. Lett., 2000, 2, 3865; CrossRef A. C. Bajji, M. Sundaram, D. G. Myszka, and D. R. Davis, J. Am. Chem. Soc., 2002, 124, 14302; CrossRef Y. Bilbille, F. A. Vendeix, R. Guenther, A. Malkiewicz, X. Ariza, J. Vilarrasa, and P. F. Agris, Nucleic Acids Res., 2009, 37, 3342. CrossRef
12. W. M. Ching, A. J. Wittwer, L. Tsai, and T. C. Stadtman, Proc. Natl. Acad. Sci. USA, 1984, 81, 57; CrossRef A. J. Wittwer, L. Tsai, W. M. Ching, and T. C. Stadtman, Biochemistry, 1984, 23, 4650; CrossRef A. J. Wittwer and T. C. Stadtman, Arch. Biochem. Biophys., 1986, 248, 540; CrossRef I. Y. Kim and T. C. Stadtman, Proc. Natl. Acad. Sci. USA, 1995, 92, 7710; CrossRef B. Nawrot, E. Sochacka, and M. Düchler, Cell. Mol. Life Sci., 2011, 68, 4023. CrossRef
13. T. Mizutani, T. Watanabe, K. Kanaya, Y. Nakagawa, and T. Fujiwara, Mol. Biol. Rep., 1999, 26, 167; CrossRef H. Mihara, S. Kato, G. M. Lacourciere, T. C. Stadtman, R. A. Kennedy, T. Kurihara, U. Tokumoto, Y. Takahashi, and N. Esaki, Proc. Natl. Acad. Sci. USA, 2002, 99, 6679; CrossRef D. Su, T. T. Ojo, D. Söll, and M. J. Hohn, FEBS Lett., 2012, 586, 717. CrossRef
14. M. Kogami and M. Koketsu, Org. Biomol. Chem., 2015, 13, 9405. CrossRef
15. (a) D. S. Wise and L. B. Townsend, J. Heterocycl. Chem., 1972, 9, 1461; CrossRef (b) C. Y. Shiue and S. H. Chu, J. Org. Chem., 1975, 40, 2971; CrossRef (c) H. Sun, J. Sheng, A. E. Hassan, S. Jiang, J. Gan, and Z. Huang, Nucleic Acids Res., 2012, 40, 5171; CrossRef (d) R. Atanu, R. C. Christopher, A. D. Fougerolles, and W. F. Andrew, US. Pat., 075177 (2013); (e) P. Bartos, A. Maciaszek, A. Rosinska, E. Sochacka, and B. Nawrot, Bioorg. Chem., 2014, 56, 49. CrossRef
16. H. G. Mautner, J. Am. Chem. Soc., 1956, 78, 5292. CrossRef
17. A. El-Tayeb, A. Qi, R. A. Nicholas, and C. E. Müller, J. Med. Chem., 2011, 54, 2878. CrossRef
18. R. Dach, J. J. Song, F. Roschangar, W. Samstag, and C. H. Senanayake, Org. Process Res. Dev., 2012, 16, 1697. CrossRef
19. I. Okamoto, K. Shohda, K. Seio, and M. Sekine, J. Org. Chem., 2003, 68, 9971. CrossRef
20. G. H. Hakimelahi, Z. A. Proba, and K. K. Ogilvie, Can. J. Chem., 1982, 60, 1106. CrossRef
21. B. M. Trost and C. G. Caldwell, Tetrahedron Lett., 1981, 22, 4999; CrossRef E. J. Corey and P. B. Hopkins, Tetrahedron Lett., 1982, 23, 4871; CrossRef K. Furusawa, K. Ueno, and T. Katsura, Chem. Lett., 1990, 97. CrossRef
22. T. Ogata, T. Shimazaki, T. Umemoto, K. Shinya, T. Ohtsuki, T. Suzuki, and T. Wada, J. Org. Chem., 2009, 74, 2585. CrossRef