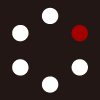
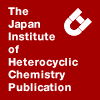
HETEROCYCLES
An International Journal for Reviews and Communications in Heterocyclic ChemistryWeb Edition ISSN: 1881-0942
Published online by The Japan Institute of Heterocyclic Chemistry
e-Journal
Full Text HTML
Received, 8th January, 2016, Accepted, 3rd February, 2016, Published online, 10th February, 2016.
DOI: 10.3987/COM-16-13404
■ Synthesis and in vitro Antitumor Activity of 9-Hydroxyellipticine Derivatives with Glucose Conjugation via Triazolylmethyl Succinate Linker
Naoya Sato, Yu Kawai, Yosuke Akaba, Shoji Honma, Norio Sakai, and Takeo Konakahara*
Department of Pure and Applied Chemistry, Faculty of Science and Technology, Tokyo University of Science, 2641 Yamazaki, Noda, Chiba 278-8510, Japan
Abstract
Three types of novel 9-hydroxyellipticine derivatives 7a-c with glucose conjugation, linked by a triazolylmethyl succinic ester bond, were synthesized, and their cytotoxicity against HeLa S-3 and 293T cells was evaluated together with ellipticine (1) and 9-hydroxyellipticine (3). These new compounds 7a-c exhibited potent antitumor activity against HeLa S-3 and 293T, and water solubility of 7a was greatly higher than those of 1 and 3. The effects of an -OCH2CH2- spacer and the amino sugar moiety on the antitumor activity were examined using 7b,c. Furthermore, one of these glucose-conjugates 7a was applied to hydrolysis, catalyzed by carboxyesterase, leading to the formation of 3 under physiological conditions.INTRODUCTION
A great deal of interest has been generated by indole alkaloids like ellipticines1 and β-carbolines2 because of their intercalation, antitumor properties, anti-HIV activities and inhibition of RNA polymerase I transcription.1b Ellipticine (1) was first identified in 1959 as a compound in the leaves of a small tropical evergreen Ochrosia sandwicensis A. DC. and Ochrosia elliptica Labill3 (see Figure 1). Ellipticine and its derivatives cause the selective inhibition of p53 protein phosphorylation in several human cancer cell lines,1 and it correlates with their cytotoxic activity. These compounds also uncouple mitochondrial oxidative phosphorylation, thereby disrupting the energy balance of cells. Further, they are known to react with DNA by an intercalation process,4a which may account for their cytotoxicity, and the inhibition of DNA topoisomerase II activity.4b We have reported the interaction mode of ellipticine and its derivatives with DNA using deflection spectroscopy.5 Ellipticine (1) is a good intercalator and an antitumor agent like derivatives of β-carboline (2).6 The unique structural features, limited toxic side effects, and complete lack of hematological toxicity of these compounds have prompted chemists to study their synthesis of a number of analogues for pharmacological evaluation.
The total synthesis of ellipticine itself was first achieved by Woodward et al. in 1959,7 and since then ellipticine and its derivatives have been synthesized by many groups. Previously, we have developed a novel and efficient method for the synthesis of ellipticine (1) via Suzuki-Miyaura coupling reaction.8 Several simple structural modifications to ellipticine derivatives have resulted in compounds with increased antitumor activity. A low level of water solubility at physiological pH, as well as systemic toxicity, has prevented the use of ellipticine (1) as a therapeutic agent. The introduction of a positive charge in the molecule improved water solubility and made a profound difference in the biological activity. Recently, we have reported the syntheses of ellipticin-2-ium and 9-hydroxyellipticin-2-ium salts along with their 5-ethoxycarbonyl analogs and their antitumor activity.9 These compounds exhibited potent antitumor activity against the human cervical cancer cell line HeLa S-3 and higher solubility in water than ellipticine itself. Because of their positive charge, the cellular uptake of these compounds, however, is affected by the membrane potential,10,11 and is expected to influence their membrane permeability. Another problem was noted when these compounds showed low selectivity against tumor cells. An alternative method to resolve this discrepancy is to introduce hydrophilic substituents like a glucose or polyethylene glycol moiety into the molecule. Chen et al. have improved water-solubility, cell-selectivity, and membrane permeability of paclitaxel prodrugs via the introduction of a glycan moiety. This modification has promoted the targeted delivery of this drug to cancer cells via glucose transporters (GLUTs).12 In medicinal chemistry, the water solubility and lipophilicity of a drug is considered to be important molecular parameters determining the absorption, the bioavailability and, sometimes, the bioactivity,13,14 and it has been reported that a therapeutic agent must be relatively soluble in water in order to pass through biological membranes.14 There were few reports on the glucose-conjugates of ellipticine (1) although many analogous reports on the acceleration of drug activity by glycan-conjugation have been published.
Herein, we focus on the development of new uncharged ellipticine derivatives that will have greater solubility in water via modification of the carbon atom at the 9-position with glucose conjugation (Figure 2). In addition, we examined the efficiency of these new drugs as potent antitumor agents with improvements in the binding ability that was related to cytotoxic activity, with evaluation by an MTT assay against HeLa S-3 and 293T cells.
RESULTS AND DISCUSSION
In a previous paper, we noted that the introduction of a 9-OH group enhanced the antitumor activity of ellipticine, and that the elongation of the tether had a tendency to suppress antitumor activity.9 For this reason, we designed 9-hydroxyellipticine derivatives with glucose conjugation at the 9-OH group (Figure 2). An ellipticine nucleus was linked with a glucose moiety by both a succinic ester bond and a triazole ring-spacer. We considered that this target compound was obtained in a high yield from the corresponding glucose azide and the ellipticin-9-yl propargyl succinate by a click chemistry (Huisgen reaction) in the presence of a Cu(I) catalyst. The compound is expected intravitally to release 9-hydroxyellipticine (3) through the action of a carboxylic ester hydrolase in cells.15
9-Hydroxyellipticine (3) was synthesized by a previously reported method,9 and then treated with monopropargyl succinate (4)16 leading to the formation of ellipticin-9-yl propargyl succinate (5) in a 78% yield under the DCC-DMAP-MS 4A conditions in a mixed solvent of dichloromethane (DCM) and DMF at room temperature (rt) for 24 h, as shown in Scheme 1.
First, a reaction of ellipticin-9-yl propargyl succinate (5) and 2-azidoethyl β-D-glucopyranoside (6a) was performed (Table 1). When a mixture of 5 and 6a was stirred in the presence of copper(II) sulfate (0.3 equiv) and sodium ascorbate (1.2 equiv) in a mixture of DMSO and H2O (10:1) at rt for 72 h, a small amount of the objective product 7a was obtained (a 15% yield) accompanied by a great deal of unreacted starting materials (entry 1). Therefore, the reaction temperature was elevated to 60 oC. However, only a trace of the product was obtained from the complex reaction mixture (entry 2). Next, although copper(I) iodide was used instead of copper(II) sulfate/sodium ascorbate at rt, the yield remained low (entry 3). The addition of a stoichiometric amount of an amine base such as Et3N and diisopropylethylamine (DIPEA) reportedly accelerates the Huisgen reaction.17 When an equimolar amount of DIPEA was added to the reaction mixture (entry 4), the starting material was completely consumed in less than 1 h of the reaction time leading to the formation of 7a with a drastically improved yield (56%). The yield, however, was by no means satisfactory. This moderate yield may have been caused by decomposition of the product, while DMSO as a solvent was removed in vacuo at about 60 oC. Indeed, 7a was obtained in a quantitative yield (92%) if the reaction mixture was directly loaded on the top of the silica gel column to remove DMSO (entry 5). As a result, we decided to use entry 5 as the optimal conditions.
It has been reported that the length of an ethylene glycol-linker changed the antitumor activity of podphyllotoxin derivatives in a subtle way,18 and that the β-D-glucosamine derivatives showed good antitumor activity.19 Therefore, in order to clarify the effects of the -OCH2CH2- spacer and the amino sugar moiety on the antitumor activity in our ellipticine system, we planed to synthesize two types of sugar-conjugated 9-hydroxyellipticine derivatives 7b,c under the optimal conditions. The reaction of 5 with β-D-glucopyranosyl azide (6b) and 2-acetamido-2-deoxy-β-D-gluco- pyranosyl azide (6c) gave the corresponding objective products 7b,c in 94 and 90% yields, respectively (Scheme 2).
Subsequently, we evaluated the cytotoxicity of the glucose-conjugates of 9-hydroxyellipticine 7a-c, ellipticine (1) and 9-hydroxyellipticine (3) on the human cervical cancer cell line HeLa S-3 and on the human embryonic kidneys (HEK) cell line 293T. We used an MTT assay to evaluate the cytotoxicity. The IC50 values of all compounds are given in Table 2.
First, the in vitro antitumor activity of the glucose-conjugated 9-hydroxyellipticine derivative 7a against HeLa S-3 was compared with those of ellipticine (1) and 9-hydroxyellipticine (3). The IC50 value of 7a was 0.50 μM (entry 1), which was smaller than any of the mother compounds, ellipticine (1; IC50 = 2.1 μM) and 9-hydroxyellipticine (3; IC50 = 1.6 μM); that is to say, the antitumor activity of 7a is enhanced 3- to 4-fold that of 3 and 1 in spite of the elongated tether. As previously reported, the elongation of the tether had a tendency to suppress antitumor activity of ellipticine derivatives.9 Therefore, this opposite effect may be explained by the accelerated delivery of 7a into the cells by the glucose-conjugation. Although the power of enhancement (3- to 4-fold) in the antitumor activity of 7a is not always high, the IC50 value of 0.50 μM for 7a is noteworthy if we take account of the suppression in the activity caused by the elongated tether. As reported for pacritaxel,12 introduction of a glucose moiety is useful to increase the uptake of glucose derivatives with bulky parent structures.
Second, the effect of the length of the linker (entry 2, 7b) on the antitumor activity was evaluated. In contrast to the antitumor activity of podphyllotoxin derivatives,18 the antitumor activity of 7b against HeLa S-3 remained strong (IC50 = 0.42 μM) in spite of a lack of the -OCH2CH2- spacer (Figure 2, n = 0). Furthermore, the 2-acetamido group (entry 3, 7c) did not affect the corresponding antitumor activity (IC50 = 0.74 μM).19 T. Honda et al. reported that the L-arabinopyranoside was the most promising antitumor agent of a series of 9-hydroxyellipticin-2-ium glycosides.20 The compounds, however, regrettably have a positive charge, caused by glycosidation on the N2 atom of ellipticine (1), in spite of its attractive property. Although the glucose moiety and the positive charge accelerates the water solubility of the compound, the latter induced both the decay of the antitumor activity10 and the cell membrane permeability.11 We have reported antitumor activity of many kinds of novel 2-alkyl-5-methoxy- carbonyl-11-methyl-6H-pyrido[4,3-b]carbazol-2-ium and 2-alkylellipticin-2-ium chloride derivatives. Their IC50 values against HeLa S-3 cells varied from 2.90 μM to 75.3 μM with the structure of the substituent on the N2 atom.9 The in vitro antitumor activity of the present 9-hydroxyellipticine glycosides are higher than those of the ellipticin-2-ium derivatives by ten-fold to two hundred-fold. On the other hand, IC50 values of compounds 7a, 7b, 1 and 3 for 293T (IC50 = 0.28, 0.298, 1.5 and 1.2 μM, respectively) were almost same as those against HeLa S-3 (IC50 = 0.50, 0.42, 2.1 and 1.6 μM, respectively).21
Next, in order to clarify whether the glucose-conjugated elliticine derivative 7a is hydrolyzed by the action of an enzyme or not, 7a was treated with carboxyesterase (from a porcine liver) in a phosphate buffer (PBS, pH 7.4), including 1% DMSO, at 37 oC. The time course of the absorbance is shown in Figure 3. The absorbance of 7a at 296 nm decreased gradually with the reaction time. If the phenolic ester bond of 7a was hydrolyzed, the increasing absorption bands of 9-hydroxyellipticine (3) should be appeared at 445-450 nm.22 Any increasing absorption band of the hydrolysates of 7a, however, was unable to find in the spectra of the reaction mixture (Figure 3). Alternatively, a colorless precipitate was appeared in the reaction mixture during the reaction. The precipitate, collected by filtration after the reaction completion, was composed from two components by HPLC/ESI-MS analysis. The major product was identified as 3 by high resolution ESI-MS. An observed exact mass of the molecular ion [M + H]+ of 3 was 263.11849 [error for C17H15N2O: +0.05 millimass unit (mmu)] and that of the minor product was 523.21496 (see Experimental). The yield of 3 was 18.5% (by HPLC/ESI-MS), and another possible hydrolysate ellipticin-9-yl succinate (8) was not detected in the reaction mixture by HPLC/ESI-MS analysis. When 3 was treated under the same conditions, the corresponding oxidative dimer was formed as reported previously.23,24 In this control reaction, an observed exact mass of the molecular ion [M + H]+ of the dimer product was 523.21324 (error for C34H27N4O2: -0.16 mmu), which was in agreement with that of the minor product from 7a within the margin of error.
Therefore, we concluded that the minor product was the dimer of 9-hydroxyellipticine (3).25 In the absence of the esterase, 7a was not reacted at all even after 3.5 h in the reaction for comparison. These facts strongly suggest that 7a works as a prodrug leading to the formation of 3 by enzymatic hydrolysis under physiological conditions in accordance with our expectation.
Finally, we determined the water solubility of 7a, as a typical example. The concentration of a saturated solution of the compound 7a in PBS was 0.77 mg/mL (see Experimental), while ellipticine (1) and 9-hydroxyellipticine (3) were only minutely soluble in water. This significant increase of the water solubility of 7a is noteworthy. It is not far behind from those of paclitaxels with glycan-conjugation,12 although we cannot simply compare the solubility of two kinds of compounds. The water solubility of drugs without a positive charge is generally improved by introduction of a hydrophilic substituent like a glucose or polyethylene glycol moiety as described above. In relation to the present study, synthesis and evaluation of antitumor activity of ellipticine derivatives possessing an oligoethylene glycol tether are in progress.
CONCLUSION
We succeeded in developing the simple and efficient synthesis of three novel 9-hydroxyellipticine derivatives 7a-c, linked with a glucose moiety by a triazole ring-succinate tether at the 9-position of an ellipticine nucleus. The glucose moiety was introduced via the Huisgen reaction. The in vitro antitumor activity of these compounds was evaluated by an MTT assay on human cervical cancer cell line HeLa S-3 and on human embryonic kidney (HEK) cell line 293T. These compounds exhibited potent antitumor activity for both cell lines. The introduction of glucose conjugation at the 9-position enhanced its solubility in water compared with those of ellipticine alone. Furthermore, 7a was hydrolyzed by esterase to generate 9-hydroxyellipticine (3) under physiological conditions. The results shown in this paper strongly suggest that the 9-hydroxyellipticine derivatives 7a-c must work as prodrugs. This is the first report of the synthesis and evaluation of the antitumor activity of the uncharged glucose-conjugates of 9-hydroxyellipticine (3) with increased water solubility.
EXPERIMENTAL
Materials and methods
All chemicals including glucose-azides 6a-c were purchased from Tokyo Chemical Industry and used without further purification, unless otherwise noted. Monopropargyl succinate (4) was synthesized from succinic anhydride and propargyl alcohol by the reported methods.16 3-(4,5-Dimethylthiazol-2-yl)- 2,5-diphenyltetrazolium bromide (MTT) was purchased from Nacalai Tesuque. Solvents were basically dried and distilled before use. Reaction progress and compound purity were monitored using thin-layer chromatography (TLC) with hexane-ethyl acetate as the irrigating system and UV light at shorter wavelengths as the visualizer. Flash chromatography was performed using Silica gel 60 (Merck). Carboxy esterase (EC 3.1.1.1; E2884; containing 6100 units of enzyme per mL) was obtained from Sigma as a suspension in a 3.2 M (NH4)2SO4 solution (pH 8). Melting points were determined using a Yanaco micro- melting-point apparatus, and the uncorrected values were reported. The 1H and 13C NMR spectra were recorded using a JEOL JNM ECP-500 (500 and 125 MHz) and a JEOL JNM ECP-300 (300 and 75 MHz) spectrometer, respectively. The 1H and 13C chemical shifts were referenced to TMS (δ 0.00) using the solvent residual peak as a reference, and the J values are reported in Hz. The low-resolution (MS) and high-resolution mass spectra (HRMS) were recorded on a JEOL JMS-MS 700 (FAB) mass spectrometer, using xenon (6 kV, 10 mA) as the fast atom bombardment gas, and a JEOL JMS-T100CS (ESI) mass spectrometer. A quantitative and qualitative HPLC/MS analysis were determined on the JEOL JMS-T100CS equipped with a Agilent 1200 HPLC apparatus with a ZORBAX Eclipse XDB-C18 column (1 x 30 mm, 3.5 mm) eluting with MeOH (0.05 mL/min) at 40 oC. Absorbance of MTT formazan was measured on a CORONA MTP-500 microplate reader.
Synthesis of ellipticine (1)
The method for the synthesis of ellipticine (1) was principally same as a previously reported method for 6-methylellipticine.9 A usual Pomeranz-Fritsch reaction was modified to give higher yield.
Pomeranz-Fritsch type reaction (a modified step-wise method): A solution of 1,4-dimethyl-3- formylcarbazole (500 mg, 2.2 mmol) in benzene (15 mL) and 2,2-diethoxyethylamine (330 mg, 2.5 mmol) was placed in a flask. The mixture was refluxed for 2 h. After the reaction, the solvent was evaporated under the reduced pressure. MeOH (15 mL) was added to the residue. NaBH4 (800 mg, 22 mmol) was then added slowly, and the reaction mixture was stirred at rt for 2 h. The solvent was evaporated under the reduced pressure. The residue was extracted with CHCl3 and dried over Na2SO4, and concentrated under reduced pressure. A solution of the residue in a mixture of MeCN (10 mL) and pyridine (1 mL) was placed in a flask. After the solution was stirred at rt for 0.5 h, 2-nitrobenzenesulfonyl chloride (1.0 g, 4.5 mmol) was added, and the reaction mixture was stirred at rt for 24 h. After the reaction, the solvent was evaporated under reduced pressure. The crude product was recrystallized from MeOH, affording N-(2,2-diethoxyethyl)-N-((1,4-dimethyl-9H-carbazol-3-yl)methyl)-2-nitrobenzene- sulfonamide (85%, 1.0 g); yellow solid; mp 175.0-176.0 ºC; 1H NMR (500 MHz, CDCl3) δ 1.46 (6H, t, J = 7 Hz), 2.41 (3H, s), 2.73 (3H, s), 3.41-3.46 (4H, m), 3.61-3.64 (2H, m), 4.56 (1H, t, J = 5.5 Hz), 4.88 (2H, s), 7.07 (1H, s), 7.21-7.26 (1H, m), 7.36-7.42 (2H, m), 7.47 (1H, d, J = 8 Hz), 7.52 (1H, d, J = 8 Hz), 7.60 (1H, d, J = 8 Hz), 7.77 (1H, d, J = 8 Hz), 8.03 (1H, brs), 8.15 (1H, d, J = 8 Hz); 13C NMR (CDCl3, 125 MHz) δ 15.3, 15.9, 16.5, 49.6, 50.6, 63.4, 102.2, 110.6, 116.8, 119.5, 121.9, 122.8, 123.7, 123.8, 124.3, 125.1, 128.7, 130.4, 130.8, 131.1, 132.8, 134.4, 138.4, 139.6, 147.8; MS (FAB+) m/z 525 [M+]; HRMS (FAB+): calcd for C27H31N3O6S (M+) 525.1933, found 525.1921.
A suspension of N-(2,2-diethoxyethyl)-N-((1,4-dimethyl-9H-carbazol-3-yl)methyl)-2-nitrobenzenesulfon- amide (500 mg, 0.95 mmol) in 6 M HCl/1,4-dioxane (1:4, 20 mL) was placed in a flask. The mixture was refluxed for 3 h. After the reaction, the reaction mixture was cooled to rt. The mixture was neutralized by the addition of aqueous sodium carbonate. The solution was extracted with CHCl3 (40 mL × 4), and dried over Na2SO4, which was removed by filtration. The filtrate was concentrated under the reduced pressure. The crude product was purified by column chromatography on silica gel [CHCl3/MeOH = 9:1 (v/v)], affording ellipticine (1) (91%, 213 mg; lit.26 40%); yellow solid; mp 309.0-310.0 ºC (lit.26 313-315 oC); 1H NMR (500 MHz, DMSO-d6), δ 2.78 (3H, s), 3.25 (3H, s), 7.25 (1H, t, J = 7 Hz), 7.51 (1H, t, J = 7 Hz), 7.56 (1H, d, J = 7 Hz), 7.91 (1H, d, J = 6 Hz), 8.37 (1H, d, 7 Hz), 8.42 (1H, d, 6 Hz), 9.69 (1H, s), 11.37 (1H, s); 13C NMR, (125 MHz, DMSO-d6) δ 11.9, 14.3, 108.0, 110.6, 115.8, 119.1, 122.0, 123.1, 123.3, 123.7, 127.0, 128.0, 132.4, 140.4, 140.5, 142.7, 149.6; MS (FAB+) m/z 247 [M + H]+.
Pomeranz-Fritsch reaction: A solution of 1,4-dimethyl-3-formylcarbazole (50 mg, 0.22 mmol) and 2,2-diethoxyethylamine (33 mg, 0.25 mmol) in benzene (20 mL) was refluxed for 2 h in a flask equipped with a Dean-Stark trap. After the reaction, the solvent was evaporated under the reduced pressure, and the crude product was recrystallized from benzene just one time to give the corresponding crude imino compound. A solution of the crude imiono compound in MeOH was added drop-by-drop to a mixture (5 mL) of H3PO4 and H2O (91:1) with stirring at 160 oC, and then stirring was continued for 10 min. After cooling to rt, H2O was added to the reaction mixture, which was then filtered. The filtrate was extracted with CHCl3 after neutralization with NH3 aq., and dried over MgSO4, which was removed by filtration. The filtrate was concentrated under the reduced pressure. The crude product was purified by column chromatography on silica gel (CHCl3), affording ellipticine (1) (28%, 15 mg; lit.26 28%).
Synthesis of 9-hydroxyellipticine (3)
The method for the synthesis of 9-hydroxyellipticine (3) was principally same as a previously reported method for 9-hydroxy-6-methylellipticine.9
A suspension of ellipticine (1) (150 mg, 0.61 mmol) and hexamethylenetetramine (1.0 g, 7.1 mmol) in TFA (25 mL) was placed in a flask. The mixture was refluxed for 30 min. After the reaction, the reaction mixture was cooled to rt. The mixture was neutralized by the addition of aqueous sodium carbonate. The mixture was extracted with CHCl3/MeOH (9:1, 50 mL × 4). The organic layers were washed with H2O. The organic layer was dried over Na2SO4 and concentrated. The solid was triturated in H2O and filtered, affording 9-formylellipticine. This product was used for the next reaction without further purification. Yield: 92% (147 mg) (lit.27 97%); yellow solid; mp 360.0 ºC (lit.27 decomp. >350 oC); 1H NMR (500 MHz, DMSO-d6), δ 2.76 (3H, s), 3.25 (3H, s), 7.64 (1H, d, J = 8.5 Hz), 7.91 (1H, d, J = 6 Hz), 8.03 (1H, d, J = 8.5 Hz), 8.46 (1H, d, J = 6 Hz), 8.83 (1H, s), 9.70 (1H, s), 10.08 (1H, s), 11.91 (1H, s); 13C NMR (125 MHz, DMSO-d6), δ 11.9, 14.3, 109.4, 111.0, 116.0, 122.3, 122.7, 123.2, 127.1, 128.1, 128.4, 128.8, 132.9, 140.7, 140.9, 146.7, 149.8, 191.9; MS (FAB+) m/z 275 [M + H]+.
A suspension of 9-formylellipticine (100 mg, 0.36 mmol) in MeOH (25 mL) was placed in a flask. The mixture was cooled to 0 ºC, and concentrated H2SO4 (100 μL) and 30% H2O2 aq were added. The mixture was refluxed for 30 min. After the reaction, the reaction mixture was cooled to 0 ºC. The mixture was neutralized by the addition of aqueous sodium carbonate. The mixture was extracted with CHCl3/MeOH (9:1, 50 mL × 2), and dried over Na2SO4, which was removed by filtration. The filtrate was concentrated under the reduced pressure. The crude product was purified by column chromatography on silica gel [CHCl3/MeOH = 9:1 to 85:15 (v/v)], affording compound 3 (69%, 66 mg; lit.27 91%); orange solid; mp 355.0 oC (lit.27 decomp. >350 oC); 1H NMR (500 MHz, DMSO-d6), δ 2.74 (3H, s), 3.20 (3H, s), 7.02 (1H, dd, J = 9, 2 Hz), 7.37 (1H, d, J = 9 Hz), 7.76 (1H, d, J = 2 Hz), 7.87 (1H, d, J = 6 Hz), 8.38 (1H, d, J = 6 Hz), 9.09 (1H, s), 9.64 (1H, s), 11.04 (1H, s); MS (FAB+) m/z 263 [M + H]+
Synthesis of ellypticin-9-yl propargyl succinate (5)
A solution of DCC (142 mg, 0.69 mmol) in CH2Cl2 (2 mL) was added to a stirred mixture of MS 4A, monopropargyl succinate (4) (107 mg, 0.69 mmol) and DMAP (17 mg, 0.14 mmol) in CH2Cl2 (8 mL) at rt. After 1 h, 9-hydroxyellipticine (3) (120 mg, 0.46 mmol) in DMF (6 mL) were added to this mixture. After 24 h, the mixture was evaporated under the reduced pressure, and extracted with CHCl3/MeOH (9:1, 100 mL × 2), washed with H2O, and dried over Na2SO4, which was removed by filtration. The filtrate was concentrated under the reduced pressure. The crude product was purified by column chromatography on silica gel [CHCl3/MeOH = 95:5 (v/v)], affording compound 5 (78%, 143 mg); yellow solid. 1H NMR (300 MHz, DMSO-d6), δ 2.78 (3H, s), 2.84 (2H, t, J = 6.5 Hz), 2.99 (2H, t, J = 6.5 Hz), 3.20 (3H, s), 3.63 (1H, t, J = 2.5 Hz), 4.82 (2H, d, J = 2.5 Hz), 7.31 (1H, d, J = 8.5 Hz), 7.60 (1H, d, J = 8.5 Hz), 7.91 (1H, d, J = 6 Hz), 8.09 (1H, s), 8.46 (1H, d, J = 6 Hz), 9.70 (1H, s), 11.46 (1H, s); 13C NMR (75 MHz, DMSO-d6), δ 11.9, 14.2, 28.6, 28.8, 52.0, 77.8, 78.4, 108.3, 110.8, 115.8, 116.4, 120.9, 121.8, 123.0, 123.2, 128.4, 132.5, 140.3, 140.5, 141.1, 143.3, 149.7, 171.4, 171.6; HRMS (FAB+), calcd for C24H21N2O4 [M + H]+: 401.1501, found 401.1488.
General procedure for the synthesis of glucose-conjugated ellipticines 7a-c (Huisgen reaction)
To a solution of CuI (17 mg, 0.089 mmol) in DMSO (0.5 mL), compound 3 (120 mg, 0.30 mmol) in DMSO (2 mL) and azide 6a-c (0.3 mmol) in a mixed solvent of DMSO (1 mL) and H2O (0.3 mL) were added. The mixture was stirred and N,N-diisopropylethylamine (DIPEA) (50 μL, 0.3 mmol) was added. After the reaction, the mixture was directly loaded onto silica gel and purified by column chromatography (CHCl3 : MeOH = 9:1 to 8:2), then the solid of the compound 7 was re-precipitated by addition of an appropriate solvent.
7a: Yield 92%; yellow solid; mp 136.0-137.0 ºC; 1H NMR (500 MHz, DMSO-d6), δ 2.78 (3H, t, J = 6 Hz), 2.80 (3H, s), 2.93 (3H, m), 3.05 (1H, m), 3.13 (2H, m), 3.21 (2H, s), 3.67 (1H, d, J = 10 Hz), 3.89 (1H, m), 4.07 (1H, m), 4.22 (1H, d, J = 10 Hz), 4.57 (3H, t, J = 5 Hz), 4.98 (1H, m), 5.02 (1H, s), 5.13 (1H, d, J = 4 Hz), 5.19 (2H, s), 7.26 (1H, dd, J = 8, 2 Hz), 7.58 (1H, d, J = 8.5 Hz), 8.09 (1H, d, J = 2 Hz), 8.25 (1H, s), 11.55 (1H, s); 13C NMR (125 MHz, DMSO-d6), δ 12.01, 14.30, 28.69, 28.89, 49.73, 57.52, 61.06, 64.86, 67.28, 70.01, 73.32, 76.60, 77.00, 79.39, 102.90, 108.54, 110.90, 116.52, 121.13, 123.22, 125.70, 128.27, 128.73, 128.93, 123.60, 140.35, 141.28, 141.51, 143.36, 149.60, 171.71, 171.85; HRMS (ESI+), calcd for C32H35N5O10Na [M + Na]+: 672.2282, found 672.2275; calcd for C32H36N5O10 [M + H]+: 650.24622, found 650.24515; UV, λmax(PBS) 296 nm (ε = 2.98 × 104 cm-1 L mol-1).
7b: Yield 94%; yellow solid; mp 145.9-146.6 ºC; 1H NMR (500 MHz, DMSO-d6), δ 2.79 (2H, t, J = 7 Hz), 2.80 (3H, s), 2.94 (2H, t, J = 7 Hz), 3.22 (3H, s), 3.44 (1H, d, J = 7.5 Hz), 3.68 (1 H, m), 3.77 (1H, m), 4.68 (1H, s), 5.22 (3H, s), 5.35 (1H, s), 5.45 (1H, d, J = 6 Hz), 5.56 (1H, d, J = 9.5 Hz), 7.29 (1H, dd, J = 9, 2.5 Hz), 7.59 (1H, d, J = 9.5 Hz), 7.97 (1H, d, J = 6 Hz), 8.11 (1H, d, J = 2.5 Hz), 8.41 (1H, s), 8.43 (1H,d, J = 6 Hz), 9.72 (1H, s), 11.62 (1H, s); 13C NMR (125 MHz, DMSO-d6), δ 12.02, 14.33, 28.64, 28.86, 60.71, 64.94, 69.53, 72.06, 76.94, 79.98, 87.49, 108.64, 110.97, 116.29, 116.55, 121.21, 121.67, 123.15, 123.28, 124.10, 128.99, 132.72, 139.40, 140.35, 141.49, 141.67, 143.41, 149.29, 171.69, 171.84; HRMS (ESI+), calcd for C30H31N5O9Na [M + Na]+: 628.2019, found 628.2013.
7c: Yield 90%; yellow solid; decomp. 178.3 ºC; 1H NMR (500 MHz, DMSO-d6), δ 1.63 (3H, s), 2.77 (2H, t, J = 6.5 Hz), 2.82 (3H, s), 2.94 (2H, t, J = 6.5 Hz), 3.23 (3H, s), 3.59 (1H, t, J = 9 Hz), 3.69 (1H, d, J = 10 Hz), 4.07 (1H, q, J = 10 Hz), 4.73 (1H, s), 5.19 (2H, s), 5.38 (2H, s), 5.73 (1H, d, J = 10 Hz), 7.31 (1H, d, J = 8.5 Hz), 7.64 (1H, d, J = 8.5 Hz), 8.01 (1H, brs), 8.11 (2H, s), 8.23 (1H, s), 8.42 (1H, brs), 9.79 (1H, s), 12.06 (1H, s); 13C NMR (125 MHz, DMSO-d6), δ 12.15, 14.53, 22.70, 28.67, 28.86, 54.41, 57.37, 60.61, 69.93, 73.85, 80.10, 86.12, 109.28, 111.34, 117.41, 121.03, 121.64, 122.85, 123.51, 123.99, 130.59, 133.10, 135.39, 140.41, 141.58, 142.60, 143.70, 147.43, 149.53, 169.23, 171.66, 171.78; HRMS (ESI+), calcd for C32H34N6O9Na [M + Na]+: 669.2285, found 669.2289.
Hydrolysis of 7a by carboxyesterase from porcine liver
Determination of time course of the hydrolysis: A carboxyesterase suspension (2 μL) was diluted with 9.9 mL of phosphate buffer (PBS, pH 7.4), which contained NaCl (8.00 g), KCl (0.20 g), Na2HPO4·12H2O (2.88 g), and KH2PO4 (0.20 g) in 1.0 L of the buffer solution. Then, 0.1 mL of the 3.52 mM stock solution of compound 7a (0.352 μmol) in DMSO was then combined with the above-mentioned buffer-diluted esterase solution. It was shaken for 30 s, and then kept in a water bath at 37 ºC with stirring. Aliquots (0.2 mL) were withdrawn from the reaction mixture after 7, 12, 22, 32, 42, 52, 62, 82, 102, 142 and 182 min and each of these samples was diluted to 3 mL with PBS and then frozen with acetone/liquid-nitrogen bath. They were analyzed by UV-Vis spectrophotometer, later. The time course for hydrolysis by the absorbance changes is shown in Figure 3.
Determination of hydrolysis product: A carboxyesterase suspension (2 μL) was diluted with 9.9 mL of PBS (pH 7.4). Aliquots (0.1 mL) of the 35.4 mM stock solution of compound 7a (3.54 μmol) in DMSO were then combined with the above-mentioned buffer-diluted esterase solution. It was shaken for 30 s, and then kept in a water bath at 37 ºC for 3 h with stirring. The reaction mixture was stood overnight at room temperature (< 10 oC) leading to the formation of precipitates, which were filtrated with a membrane-filter and collected with 3.3 mL of MeOH. The solution was applied to HPLC/ESI-MS, which showed that the precipitates were composed of two products. The major product was 9-hydroxyellipticine (3) and the minor product was a dimer of 3 as shown bellow.
9-Hydroxyellipticine (3): yield 18.5% (by HPLC/ESI-MS); HRMS (ESI+), calcd for 12C17H15N2O [M + H]+: 263.11844, found 263.11849.
The minor product; HRMS (ESI+), calcd for 12C34H27N4O2 [M + H]+: 523.21340, found 523.21496; HRMS (ESI-), calcd for 12C34H25N4O2 [M - H]-: 521.19775, found 521.19656.
Dimerization of 9-hydroxyellipticine (3): A solution of 3 (1.85 mg, 7.05 mmol) in 0.2 mL DMSO was combined with 20 mL PBS and the thus-obtained turbid solution was incubated at 37 oC for 3 h in the absence of the esterase and stood overnight at room temperature in the same way as the hydrolysis reaction of 7a mentioned above. After the reaction, the appeared precipitates were collected by filtration, and dissolved in 5 mL of a mixed solvent of CH2Cl2 and MeOH (3:2). The solution was applied to HPLC/ESI-MS. The reaction mixture contains only the dimer of 3 except for the starting material 3.
9-Hydroxyellipticine (3): recovery 43% (by HPLC/ESI-MS); HRMS (ESI+), calcd for 12C17H15N2O [M + H]+: 263.11844, found 263.11839.
The dimer of 9-hydroxyellipticine: yield 57%; HRMS (ESI+), calcd for 12C34H27N4O2 [M +H]+: 523.21340, found 523.21324.
The reaction of 3 in the presence of the esterase also afforded the same dimer. Recovery and the yield were not determined. HRMS (ESI+), calcd for 12C34H27N4O2 [M + H]+: 523.21340, found 523.21359.
Evaluation of the water solubility of 7a
A stock solution of 7a (1.5 mg/4 mL H2O) was diluted to 1/10 concentration. The obtained solution was additionally diluted to 1/2, 1/4, and 1/8 concentrations. The absorbance of each solution was determined at 296 nm to make a calibration curve. Subsequently, compound 7a (3.5 mg) was suspended in H2O (4.0 mL) and sonicated at rt to prepare a saturated solution, which was filtered to remove the undissolved 7a. An aliquot of the filtrate was diluted to the appropriate concentration and the absorbance of the solution was measured at 296 nm leading to the solubility of 7a (0.77 mg/mL).
Evaluation of Antitumor Activity
Cell lines and culture: HeLa S-3 cells were maintained in MEM (Nissui) supplemented with 10% FBS (GIBCO), 2% HEPES, 3% NEAA, 1.5% NaHCO3 (7.5 w/v% solution) and 2 mM L-glutamine in a water-saturated atmosphere of 5% CO2 at 37 oC. The 293T cells were maintained in DMEM (Nissui) supplemented with 10% FBS and 1% penicillin/streptomycin in a water-saturated atmosphere of 5% CO2 at 37 oC.
Cell viability assay: Cell viability was determined by a 3-(4,5-dimethylthiazol-2-yl)-2,5-diphenyl- tetrazolium bromide (MTT) assay, which is a method for determining cell viability by measuring the mitochondrial dehydrogenase action. Cells were seeded in a 96-well cell culture cluster (Becton Dickinson) at a density of 2 × 104 cells/mL and cultured 3 h prior to drug treatment. Cells were exposed at 37 oC for 24-72 h to ellipticine derivatives. The MTT reagent was prepared at a concentration of 2 mg/mL in Dulbecco’s PBS without calcium and magnesium, and stored at 4 oC. After treatment for the indicated times, the cells were incubated with MTT reagent for 4 h at 37 oC. The plate was centrifuged at 3000 rpm for 10 min, and the medium was removed. To solubilize the resultant MTT formazan, 200 μL/well of dimethyl sulfoxide (DMSO) was added to each well followed by thorough mixing using a mechanical plate mixer. Absorbance at 540 nm was measured on a microplate reader, and the percentage of cell viability was taken as the percentage absorbance at 540 nm of ellipticine derivative-treated cells against control cells. IC50 values are reported as the means of three experiments and the standard deviations are given together.
ACKNOWLEDGMENTS
This work was partially supported by a Grant-in-Aid for Scientific Research from MEXT, a matching fund subsidy from MEXT 2004-2006 (No.16550148), 2009-2011 (No. 21590025) and 20012–2014 (No. 24590025); a Grant from the Japan Private School Promotion Foundation (2008-2009); and a grant for the Development of strategic Research Center in Private Universities supported by MEXT, Center for Technologies against Cancer (CTC), 2009-2013. We would like to thank Dr. Tomoko Okada (Institute for Biological Resources and Functions, National Institute of Advanced Industrial Science and Technology) for kindly providing the HeLa S-3 cells and Mrs. Hitomi Isoda (Research Equipment Center, Tokyo University of Science) for HPLC/ESI-MS analysis.
References
1. (a) J. Y. Kim, S. G. Lee, J.-Y. Chung, Y.-J. Kim, J.-E. Park, H. Koh, M. S. Han, Y. C. Park, Y. H. Yoo, and J.-M. Kim, Toxicology, 2011, 289, 91; CrossRef (b) W. J. Andrews, T. Panova, C. Normand, O. Gadal, I. G. Tikhonova, and K. I. Panov, J. Biolog. Chem. 2013, 288, 4567.
2. J. Wu, G. Cui, M. Zhao, C. Cui, and S. Peng, Mol. Biosyst., 2007, 3, 855. CrossRef
3. S. Goodwin, A. F. Smith, and E. C. Horning, J. Am. Chem. Soc., 1959, 81, 1903. CrossRef
4. (a) N. C. Garbett and D. E. Graves, Current Med. Chem. Anti-cancer Agents, 2004, 4, 149; CrossRef (b) M. Stiborová, M. Stiborova-Rupertova, L. Borek-Dohalska, M. Wiessler, and E. Frei, Chem. Res. Toxicol., 2003, 16, 38. CrossRef
5. N. Morii, G. Kido, T. Konakahara, and H. Morii, J. Phys. Chem. B, 2005, 109, 15636. CrossRef
6. R. Ikeda, T. Kimura, T. Tsutsumi, S. Tamura, N. Sakai, and T. Konakahara, Bioorg. Med. Chem. Lett., 2012, 22, 3506. CrossRef
7. R. B. Woodward, G. A. Iacobucci, and F. A. Hochstein, J. Am. Chem. Soc., 1959, 81, 4434. CrossRef
8. T. Konakahara, Y. B. Kiran, Y. Okuno, R. Ikeda, and N. Sakai, Tetrahedron Lett., 2010, 51, 2335. CrossRef
9. R. Mori, A. Kato, K. Komenoi, H. Kurasaki, T. Iijima, M. Kawagoshi, Y. B. Kiran, S. Takeda, N. Sakai, and T. Konakahara, Eur. J. Med. Chem., 2014, 82, 16. CrossRef
10. J.-Y. Charcosset, A. Jacquemin-Sablon, and J.-B. Le Pecq, Biochem. Pharm., 1984, 33, 2271. CrossRef
11. A. K. Larsen, J. Paoletti, J. Belehradek, and C. Paoletti, Cancer Res., 1986, 46, 5236.
12. Y. S. Lin, R. Tungpradit, S. Sinchaikul, F. M. An, D. Z. Liu, S. Phutrakul, and S. T. Chen, J. Med. Chem., 2008, 51, 7428. CrossRef
13. D. Thibeault, C. Gauthier, J. Legault, J. Bouchard, P. Dufour, and A. Pichette, Bioorg. Med. Chem., 2007, 15, 6144. CrossRef
14. C. G. Wermuth, ‘The practice of medicinal chemistry,’ 3rd ed., Academic Press, 2011, pp. 767-785.
15. Tsujihara et al. have reported that 9-acyloxyellipticines exhibited good antitumor activity against several tumors in mice and released 3 in a body; N. Harada, K. Ozaki, K. Oda, N. Nakanishi, M. Ohashi, T. Hashiyama, and K. Tsujihara, Chem. Pharm. Bull., 1997, 45, 1156.
16. P. Antoni, Y. Hed, A. Nordberg, D. Nyström, H. von Holst, A. Hult, and M. Malkoch, Angew. Chem. Int. Ed., 2009, 48, 2126. CrossRef
17. B. H. Lipshutz and B. R. Taft, Angew. Chem., 2006, 118, 8415. CrossRef
18. C. T. Zi, F. Q. Xu, G. T. Li, Y. Li, Z. T. Ding, J. Zhou, Z. H. Jiang, and J. M. Hu, Molecules, 2013, 18, 13992. CrossRef
19. F. C. Giuliani and N. O. Kaplan, Cancer Res., 1980, 40, 4682.
20. T. Honda, M. Inoue, M. Kato, K. Shima, and T. Shimamoto, Chem. Pharm. Bull., 1987, 35, 3975. CrossRef
21. Ellipticin-9-yl methyl succinate also exhibited potent antitumor activity, and its cell cytotoxicity for 293T (IC50 = 2.24 μM) was approximately 7-fold smaller than that for HeLa S-3 (IC50 = 0.31 μM). The succinate was synthesized in a 69% yield as yellow solid by the same method as 5; 1H NMR (500 MHz, DMSO-d6), δ 2.67 (3H, s), 2.74 (2H, t, J = 6.5 Hz), 2.92 (2H, t, J = 6.5 Hz), 3.09 (3H, s), 3.66 (3H, s), 7.25 (1H, dd, J = 8.5, 2 Hz), 7.53 (1H, d, J = 9 Hz), 7.80 (1H, d, J = 6 Hz), 8.00 (1H, s), 8.38 (1H, d, J = 6 Hz), 9.61 (1H, s), 11.36 (1H, s); 13C NMR (125 MHz, DMSO-d6), δ 11.8, 14.0, 28.6, 28.9, 51.6, 108.3, 110.7, 115.8, 116.3, 120.9, 121.8, 122.9, 123.2, 128.3, 132.5, 140.3, 140.5, 141.0, 143.3, 149.6, 171.7, 171.5; HRMS (FAB+), calcd for C22H21N2O4 [M + H]+: 377.1501, found 377.1490.
22. It may be considered that the lack of the absorption maximum of 9-hydroxyellipticine (3) at 445-450 nm in Figure 3 was caused by the low water-solubility of 3, the formation of the dimer of 3, and their precipitation. UV spectrum of 3 reportedly exhibited one of the absorption maxima at 445 nm ( = 3.2 × 103 cm-1 L mol-1 in DMSO), which was assignable to the corresponding anionic forms (C. Auclair and C. Paoletti, J. Med. Chem., 1981, 24, 289), and that of 9-hydroxy-2-methylelliptcin- 2-ium chloride exhibited absorption maxima at about 295 nm ( > 104), 364 ( = 4.5 × 103), 378 (4.7 × 103), and 449 nm (2.6 × 103 cm-1 L mol-1) in phosphate buffer (pH 7.40) containing 0.l M leucine, just for reference (C. Auclair, Photochem. Photobiol., 1987, 45, 35 and references cited therein).
23. J. Moiroux and A. M. Armbruster, J. Electroanal. Chem. Interfacial Electrochem., 1984, 165, 231. CrossRef
24. M. D. Disney, B. Liu, J. L. Childs-Disney, and W.-Y. Yang, WO, 2014, 036395.
25. Although the structure of the dimerized 3 is not clear at present time, it may be analogous to that of phenols, which oxidatively-dimerize to give ortho-ortho and ortho-para coupled products as the predominant C-C dimers; D. R. Armstrong, R. J. Breckenridge, C. Cameron, D. C. Nonhebel, P. L. Pauson, and P. G. Perkins, Tetrahedron Lett., 1983, 24, 1071.
26. L. Dalton, S. Demerac, B. Elmes, J. Loder, J. Swan, and T. Teitei, Aust. J. Chem., 1967, 20, 2715. CrossRef
27. J. P. M. Plug, G. J. Koomen, and U. K. Pandit, Synthesis, 1922, 1221.