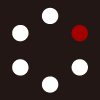
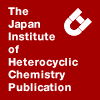
HETEROCYCLES
An International Journal for Reviews and Communications in Heterocyclic ChemistryWeb Edition ISSN: 1881-0942
Published online by The Japan Institute of Heterocyclic Chemistry
e-Journal
Full Text HTML
Received, 21st June, 2016, Accepted, 17th August, 2016, Published online, 6th September, 2016.
■ Exploring the Synthesis of Deceptively Simple Biginelli Products through N-CN Bond Cleavage
Juan Jesús Nolasco Fidencio, Hulme Ríos-Guerra,* Gilberto González-Villanueva, José Guillermo Penieres-Carrillo, Gustavo Guevara Balcázar, Ma. Inés Nicolás-Vázquez, Hulme Ríos-Guerra, and Francisco Delgado
Faculty of Higher Cuautitlan Studies, Chemistry Science Department, UNAM, Av. 1° de Mayo s/n Sta. Ma. Gpe. las Torres, Postcode 54740, Cuautitlán Izcalli, Mexico
Abstract
Brønsted-Lowry acid-promoted cleavage of the N-CN bond in 2-cyanoimino-3,4-dihydro-(1H)-pyrimidine is described. Formic acid efficiently activates cyclic N-cyanoguanidines through an O-formylisourea reactive intermediate, leading to chemospecific N-CN bond cleavage of the cyanoimine moiety. This low environmental-load method provides step- and atom-economical access to difficult-to-obtain acid-sensitive Biginelli products in excellent yields.INTRODUCTION
Cyanamides (R1R2N-CN) and cyanoimines (R1R2C=N-CN) are valuable building blocks in organic chemistry.1 Although many reactions at the cyano group have been explored,1b methods for cleaving the N-CN bond in compounds containing an cyanamide and cyanoimine groups are rare, presumably due to extended conjugation and tautomerization effects that make it strong and not readily broken. Because of this challenge, catalytic cleavage of the N-CN bond in cyanamide using either well-designed transition metal catalysts or metal-free Lewis acids [for example, CpFe(CO2Me, CpMo(CO3Me and B(C6F5)3] has only recently been reported (Figure 1a and 1b).2 Cleavage of this bond in azaheterocycles containing an cyanoimine functional group is even rarer and sparsely documented. However, CF3CO2H and HCl are among the few known useful Brønsted-Lowry acids used to cleave the N-CN bond in N-cyanosulfoximines and N-cyanoguanidines, respectively.3 Although hydrochloric acid has been used in the preparation of some acyclic and cyclic-guanidines (Figure 1c), their major limitations result from its incompatibility with substrates having sensitivity functionality.
Accordingly, herein we present a simple and inexpensive methodology for cleaving the N-CN bond in 2-cyanoiminopyrimidine using mild Brønsted-Lowry acid. In addition, the developed methodology is used to prepare difficult-to-obtain acid-sensitive Biginelli products featuring 2-imino-3,4-dihydro-(1H)-pyrimidine salt framework 3. This skeleton has been found embedded in the structure of marine alkaloid stimulator of NO production e.g. uropocidins A (I) and B (II)].4 Thereby, this class of azaheterocycles has attracted attention as key building block for assembling structurally complex natural guanidine alkaloids (e.g. triazaacenaphthalene type III) and to synthesize small libraries of highly nitrogenous heterocycles IVa-d (Figure 1).5 To the best of our knowledge, the direct Biginelli reaction fails to produce such products. Furthermore, we explore the scope of this protocol in the preparation of 2-amino- and 2-guanidino-1,4-dihydropyrimidine compounds. Notably, this strategy avoids double Biginelli product formation6 while improving the overall yields of 3 compared to other Biginelli-like strategies, based on pyrazole carboxamidine or triazone-protected guanidine and resin-bound isothiourea Biginelli adduct.7 Most importantly, this strategy avoids the use of expensive tailored catalysts and elaborate multi-step synthetic methodologies and tedious work-up.
RESULTS AND DISCUSSION
We first examined the N-CN bond cleavage reaction using several Brønsted-Lowry acids, as outlined in Table 1. Preliminary NMR analysis of the crude reaction provided no evidence of N-CN bond cleavage when 1a8 (1 mmol) was reacted with HCl (5 mL) under Durant´s condition3a (Table 1, entry 1); rather, it revealed a complex mixture of products. In-depth spectroscopic analyses supported the ready formation of the hydration product 5a [2-(N-carbamoylamino)-1,4-dihydropyrimidine], which was isolated in 88% yield following quenching of the crude reaction with Na2CO3 (Scheme 1, Path A, step 1). These results suggest an initial efficient hydrolysis reaction, followed by a degradative hydrolytic rupture as a result of the extended reaction time and the harsh acidic conditions.
In our attempt to avoid this detrimental effect, we explored the reaction of 2-ureidopyrimidine 5a with milder organic acid.9 Thus, 5a was treated with excess formic acid for 24 h and heated at 101 °C (Scheme 1, Path A, step 2). We were pleased to find that 3,4-dihydropyrimidin-2(1H)-iminium formate 3a was formed in 90% overall yield. Spectroscopic analysis showed that the 2-exo-iminium formate tautomer core exhibited three key signals interchangeable with D2O at 11.62, 10.02 and 8.05 ppm in the 1H NMR spectrum, which were attributable to NH-1, NH-3 and =NH2protons, respectively. Unfortunately, the stringent acid conditions required for the initial hydration step became troublesome when acid-sensitive substrates were employed. Therefore, we did not further pursue this two-step procedure.
In the light of these results, we look to take advantage of formic acid’s capacity to perform hydrolysis reaction and nucleophilic addition reaction, besides its catalytic property. Likewise we envisaged that the carbamoyl group activate the 2-ureidopyrimidine under acid condition, O-formyloxy group might activate Nsp3-Csp2 bond cleavage of -NCOCHO=NH-type O-formylisoureapyrimidine while incorporating the hydrolytic reaction and catalyst effects induced by the formic acid. Accordingly, in situ generation of either 2-ureidopyrimidine or O-formylisoureapyrimidine species could lead to the preparation of 3a in a one-step process.
Consequently, 1a was treated with an excess of formic acid and heated at ~101 °C for an extended period while monitoring the reaction using NMR spectroscopy. Remarkably, under these conditions, we did not find evidence of any hydration products. Rather, the O-formylisoureapyrimidine intermediate 2a, N-(1,4-dihydropyrimidin-2-yl)carbamimidic formic anhydride (Scheme 1, Path B, step 3), was detected and readily isolated in 95% overall yield (Table 1, entry 2). The NMR spectroscopy data were consistent with the proposed structure. Briefly, the key NH-aza-enol formate moiety appears as three singlet signals at 8.24, 7.04 and 6.33 ppm, in addition to two signals occurring at 162.7 (-NHC=NHO-) and 165.3 ppm (HCO2-) in the 1H and 13C NMR spectra, respectively.
Further analysis of the reaction mixture at 2, 4 and 16 h revealed 4:1, 1:1 and 1:17 mixtures of 2a and 3a, respectively; the highest transformation was reached at 24 h. Afterwards, 3a was readily precipitated with a Na2CO3-saturated solution at pH 3-4 and recovered in 90% yield (Table 1, entry 2). Moreover, the corresponding free-form, 2-amino-1,4-dihydropyrimidine 6a-b, was obtained in high yield at pH 10 (Scheme 2). This approach substantially improved the yield of the 2-imino-3,4-dihydropyrimidine salt compared with aminolysis of resin-bound isothiourea Biginelli adducts (63%) and triazone-protected guanidine Biginelli (53-76%) strategies.7
Notably, by-products such as the overreduction product 7a and rearrangement products 8a of the O-formylisoureapyrimidine 2a were not detected under this protocol.10 It is worth noting that because 2a exhibits higher reactivity in the guanylation reaction than 1a, it is well suited for synthesizing guanidine derivatives that are difficult to access directly from the Biginelli reaction. For example, 2-guanidino-1,4-dihydropyrimidine 9a was readily obtained upon reaction with NH4OH (Scheme 1).11 Thus, this method offer an alternative with regard to the preparation of novel molecular ligands of pharmacological interest.5 On the other hand, stronger Brønsted-Lowry acids such as CF3CO2H resulted in an ineffective acid promoter, whereas weaker acids such as MeCO2H afforded the corresponding 3,4-dihydropyrimidin-2(1H)-iminium acetate 4a, albeit in lower yields than those achieved using HCO2H (Table 1, entries 3 and 4, respectively). After identifying suitable reaction conditions, further substrates were examined (Scheme 3). Acid-sensitive arenecarbaldehydes bearing methylenedioxy group on the aromatic ring give 3,4-dihydropyrimidin-2(1H)-iminium formate 3c in excellent yield (91%). Most importantly, this strategy has succeeded where other methods have failed.4 For example, the presence of the thiophen-2-yl group was also well tolerated and afforded the difficult-to-obtain product 3d in 85% yield. Furthermore, it appears that the electronic effects of substituent at the ortho position on the aromatic ring affected the overall yield (e. g. 3e vs 3f).
Accordingly, a reasonable mechanism was proposed, as outlined in Scheme 4. In support of the proposed mechanism, we obtained experimental evidence (vide supra) that suggest the initial formation of 2a by specific acid-nucleophilic catalysis.12 Based on carbodiimide-type mechanism we assume that 2a evolves from a carbodiimide species such as 10a after experiment a nucleophilic attack by the formate partner. Further reaction with HCO2H13 leads to the irreversible formation of the thermodynamically stable 2-imino-3,4-dihydro-(1H)-pyrimidine salt 3a, allegedly passing through specie 11a.
In summary, an environmentally benign one-pot decyanation reaction has been developed. The operational simplicity and excellent reaction efficiency, combined with its step and atom economy, make this reaction a convenient approach for synthesizing difficult-to-obtain acid-sensitive 2-imino-3,4-dihydro-(1H)-pyrimidine salt.
ACKNOWLEDGEMENTS
This work was supported in part by PIAPI-1618 research program. Technical support provided by Fernando Sotres is also strongly appreciated by the authors.
References
01. (a) D. D. Nekrasov, Russ. J. Org. Chem., 2004, 40, 1387;; CrossRef (b) D. D. Nekrasov, Chem. Heterocycl. Compd., 2005, 41, 809;; CrossRef (c) A. Dömling, E. Herdtweck, and S. Heck, Tetrahedron Lett., 2006, 47, 1745. CrossRef
02. (a) K. Fukumoto, O. Tsukuru, M. Itazaki, and H. Nakazawa, J. Am. Chem. Soc., 2009, 131, 38;; CrossRef (b) Z. Pan, S. M. Pound, N. R. Rondla, and C. J. Douglas, Angew. Chem. Int. Ed., 2014, 53, 5170;; (c) K. Ouyang, W. Hao, W. X. Zhang, and Z. Xi, Chem. Rev., 2015, 115, 12045. CrossRef
03. (a) G. J. Durant, J. C. Emmett, C. R. Ganellin, P. D. Miles, M. E. Parsons, H. D. Prain, and G. R. White, J. Med. Chem., 1977, 20, 901;; CrossRef (b) N. Barry, N. Brondel, S. E. Lawrence, and A. R. Maguire, Tetrahedron, 2009, 65, 10660;; CrossRef (c) F. Ishikawa, Y. Watanabe, and J. Saegusa, Chem. Pharm. Bull., 1980, 28, 1357. CrossRef
04. (a) B. L. Nilsson and L. E. Overman, J. Org. Chem., 2006, 71, 7706;; CrossRef (b) T. N. Makarieva, E. K. Ogurtsova, V. A. Denisenko, P. S. Dmitrenok, K. M. Tabakmakher, A. G. Guzii, E. A. Pislyagin, A. A. Eskov, V. M. Kozhemyako, D. L. Aminin, Y. Wang, and V. A. Stonik, Org. Lett., 2014, 16, 4292. CrossRef
05. D. M. Arnold, M. G. La Porte, S. M. Anderson, and P. Wipf, Tetrahedron, 2013, 69, 7719. CrossRef
06. R. Milcent, J.-C. Malanda, G. Barbier, and J. Vaissermann, J. Heterocycl. Chem., 1997, 34, 329. CrossRef
07. (a) See reference 4;; (b) O. Kappe, Bioorg. Med. Chem. Lett., 2000, 10, 49. CrossRef
08. H. Ríos, D. P. Zamora, E. J. Mota, M. A. Pastén, I. Valencia-Hernández, J. Correa-Basurto, and F. Delgado, Tetrahedron, 2008, 64, 3372. CrossRef
09. A. M. Prokhorov, D. N. Kozhevnikov, V. L. Rusinov, and O. N. Chupakhin, Russ. Chem. Bull. Int. Ed., 2003, 52, 1195. CrossRef
10. M. B. Smith, Org. Synth., McGraw-Hill, 1994, pp. 476-477.
11. The derivative chemistry of the O-formylisourea 2a will be reported in an independent study.
12. S. V. Hill, A. Williams, and J. L. Longridge, J. Chem. Soc., Perkin Trans. 2, 1984, 1009. CrossRef
13. (a) N. Menashe, D. Reshef, and Y. Shvo, J. Org. Chem., 1991, 56, 2912;; CrossRef (b) Z. Wan, D. M. Chauncey, J. Y. Pu, and T. Y. Zhang, J. Org. Chem., 2006, 71, 826. CrossRef