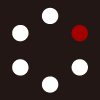
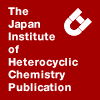
HETEROCYCLES
An International Journal for Reviews and Communications in Heterocyclic ChemistryWeb Edition ISSN: 1881-0942
Published online by The Japan Institute of Heterocyclic Chemistry
e-Journal
Full Text HTML
Received, 11th August, 2016, Accepted, 21st October, 2016, Published online, 9th November, 2016.
■ Dipolar Cycloadditions of Nitrones in Aqueous Media
Giorgio Molteni*
Department of Organic and Industrial Chemistry, University of Milano, Via Golgi 19, 20133 Milano, Italy
Abstract
1,3-Dipolar cycloadditions of nitrones in aqueous media have been recently exploited as a non-conventional protocol in the synthesis of the fully- or partly-saturated isoxazole ring. Water as the reaction medium can affect positively both cycloaddition rates and yields, while the large array of latent functionalities displayed by the cycloadducts is of interest in their further transformations. A systematic review of literature data is given; improvements with respect to the nitrone cycloaddition carried out in organic solvents will be taken into account.CONTENTS
I. Introduction
II. Intermolecular cycloadditions
III. Intramolecular cycloadditions
IV. Organocatalysed cycloadditions
I. INTRODUCTION
Although water is the solvent of choice for nature to perform its chemical transformations, the overwhelming majority of organic chemists carries out their reactions in non-aqueous media. This is a consequence of the well-known fact that water can be aggressive towards many classes of organic functionalities and intermediates, thus leading to a strict exclusion of water from reaction mixtures. On the other hand, the use of water as the reaction medium displays a number of desirable features: (i) the pH can be easily monitored,1 (ii) reaction rates can be significantly increased,2-9 (iii) product separation can often be achieved by simple filtration of the crude reaction mixture,10,11 and (iv) environmentally-friendly procedures can be successfully elaborated in some cases.12,13 In the light of these considerations it is not surprising that some general reviews14-22 and books23-26 have appeared in the last decade covering the field of water-promoted organic reactions. Among the organic transformations which appear to benefit from aqueous media, 1,3-dipolar cycloadditions occupy a prominent place and their behaviour in water has been recently reviewed.27-29 Recent papers on this subject are related to azides,30-33 nitrile oxides,34,35 and nitrilimines36-40 as a fertile source of heterocycles. Curiously enough, nitrone cycloadditions in aqueous media have received little attention in such reviews despite the large array of latent functionalities displayed by the fully- or partly-saturated isoxazole ring in the cycloadducts.41
The aim of this paper is to present the first systematic review devoted to the growing field of nitrone cycloadditions carried out in aqueous media. Improvements with respect to the cycloadditions performed in organic solvents will be taken in account as well as novel fascinating topics like micelle-promoted42 and organocatalytic nitrone cycloadditions.
II. INTERMOLECULAR CYCLOADDITIONS
Nitrones were first prepared by von Beckmann in 1890,43 and they were soon recognised as species “capable to undergo addition 1,3”.44 Seven decades later, the monumental work on 1,3-dipolar cycloadditions performed by Huisgen allowed the classification of nitrones as belonging to the family of azomethinium betaines.45-47 The behaviour of nitrones in organic solvents was then studied deeply48-50 and the issue of cycloaddition regio- and stereoselectivity was rationalised in the light of the FMO theory.51,52
It is well-known that 1,3-dipolar cycloadditions are usually concerted processes whose rate is scarcely influenced by the solvent.47 The speed-up of cycloaddition rates in water is mainly due to the hydrophobic effect,43,54 which expresses the tendency of non-polar, little water-soluble organic species to aggregate themselves in order to reduce the contact surface between water and the non-polar organic substrates. Such an effect is enforced by the volumetric contraction of the reagents along the reaction coordinate, this means that 1,3-dipolar cycloadditions which show negative activation volumes55,56 are sensitive to the hydrophobic effect.
For the sake of clarity, the presentation of intermolecular nitrone cycloadditions performed in water or in aqueous media is divided into three parts: kinetic evidences, nitrone-ethylene cycloadditions and nitrone-acetylene cycloadditions.
II.1. Kinetic evidences
The cycloadditions between C,N-diphenylnitrone and di-n-butyl fumarate or dimethyl fumarate were investigated in water, aqueous solutions and organic solvents at 65 °C.57 Second-order rate constants were determined, their values are listed in the Table 1 and deserve some comments. In organic solvents, the rate constants observed in the cycloaddition with dimethyl fumarate were higher than those reported with di-n-butyl fumarate. The authors explained this behaviour as a consequence of the larger steric demands of the bulky n-butyl group compared to the methyl one. Then, the cycloaddition rates were found to decrease with increasing polarity of the organic solvent, which was expressed through the normalised Dimroth-Reichardt parameter ETN.58 The following linear relationship between second-order rate constants and ETN was found.
The second-order rate constants as a function of R were just reversed in the case of the cycloadditions performed in water or aqueous solutions. This points to the facts that: (i) as can be supposed from their concerted nature, the nitrone-olefin cycloadditions are scarcely influenced by the solvent polarity, (ii) the dramatic speed-up of cycloaddition rates in water or aqueous solutions59 depends upon the hydrophobic effect. In fact, the presence of alkali metal salts in water markedly increased the cycloaddition rate of the more lipophilic di-n-butyl fumarate; the increase of alkyl chain lenght enhances the hydrophobicity of the fumarate giving more effective hydrophobic interactions. By contrast, the presence of urea in water incresases the solubility of non-polar organic species thus decreasing the hydrophobicity of the reactants and hence the cycloaddition rates.
Second-order rate constants of the cycloadditions between C,N-diphenylnitrone and di-n-butyl fumarate in aqueous solutions of ethanol and 1-propanol were also obtained. The increase of the cycloaddition rate at low mole fractions of water is quite slow. At higher mole fractions of water in ethanol, the cycloaddition rates increases more rapidly compared with the solutions in 1-propanol. This can be due to the better ability of 1-propanol to dissolve the organic reactants thus decreasing their hydrophobicity.
The activation parameters have been calculated in the case of the cycloaddition between C,N-diphenyl- nitrone and dimethyl fumarate in various solvents including water.57 The values listed in the Table 2 show that the activation entropy in water is positive in contrast with organic solvents. This may be due to the presence of organic aggregates which are capable to partly destroy the well-ordered structure of water. The positive sign of the TΔS≠ terminus implies higher cycloaddition rates compared to organic solvents.
II.2. Nitrone-ethylene cycloadditions
The reaction between C,N-diphenylnitrone and methyl acrylate, acrylonitrile or vinyl acetate constitutes one of the first examples of intermolecular nitrone-olefin cycloadditions in water (Scheme 2).60 As can be inferred from Table 3, the reactions performed in water gave better yields compared to benzene, although isoxazolidine cycloadducts (1) were obtained as mixtures of regio- and stereoisomers. In particular, the cycloaddition between C,N-diphenylnitrone and methyl acrylate in water at 25 °C gave a complex mixture of regio- and stereoisomeric cycloadducts (see Scheme 3) whose ratio did not change significantly in benzene. It was found that the concentration of the organic reactants suspended in water did not affect cycloaddition rates and yields. This behaviour was rationalised by the authors as the result of hydrogen bonding occurring between water and the carbonyl oxygen of the dipolarophile. Within this picture, the reactivity of the conjugated dipolarophiles should be enhanced due to the greater electrophilic charachter of the β-carbon subjected to nitrone attack.61 A similar degree of complexity was observed in the reaction of the tymine-based nitrone (2) with alkyl acrylates (Scheme 4, Table 4).62
A moderate diastereopreference to the anti-isoxazolidine (8) was observed by reacting the decanal- derived nitrone (7) with methyl- or dodecyl acrylate, as shown in Scheme 5 and Table 5.63 Both overall yields and stereoselectivity were scarcely affected from the reaction medium.
A similar behaviour in regio- and stereoselectivity was found in the case of the cycloadditions of chiral (racemic) nitrone (10) with ethyl acrylate or styrene (Scheme 6, Table 6).64-66 The outcome of the reactions carried out in water at rt is much better than that in dichloromethane.
The nitrone-olefin cycloaddition allows the obtainment of isoxazolidines with significant anti-bacterial properties.67 An example is given by the reactions between N-phenyl-C-dimethylaminonitrone (13) and several mono- or 1,2-disubstituted ethylenic dipolarophiles (Scheme 7); such cycloadditions gave the syn-isoxazolidines (14) as single regio- and stereoisomers.68 It can be inferred from Table 7 that reactions performed in water at rt required shorter times and gave better yields compared to dichloromethane.
Enantiopure nitrones (15) and (18) were synthesised from d-ribose and used as key intermediates in the synthesis of aza-C-disaccharides and modified nucleosides.69 Cycloaddition of (15) to alkyl acrylates at 80 °C gave a couple of 5-substituted enantiopure isoxazolidines anti-(16) and syn-(17) with low diastereoselectivity (Scheme 8, Table 8).69 The preferred product anti-(16) arises from the corresponding endo transition state. As far as the cyclic nitrone (18) is concerned, a similar behaviour compared to (15) was experienced at 25 °C (Scheme 9, Table 9).69
The nitrone-olefin cycloaddition allows the obtainment of isoxazolidines with significant anti-bacterial properties.67 An example is given by the reactions between N-phenyl-C-dimethylaminonitrone (13) and several mono- or 1,2-disubstituted ethylenic dipolarophiles (Scheme 7); such cycloadditions gave the syn-isoxazolidines (14) as single regio- and stereoisomers.68 It can be inferred from Table 7 that reactions performed in water at rt required shorter times and gave better yields compared to dichloromethane.
To this point, it can be perceived that the acceleration of water-nitrone cycloadditions arises from an interplay of factors. It is known that the rate of nitrone cycloadditions is slightly lowered a little by enhancing the polarity of the solvent,71,72 so that the high polarity of water73 can be ruled out as the accelerating cause. A simple but rather partial rationale may be provided on the basis of the FMO theory.74 Generally speaking, the ethylenic dipolarophiles are weak hydrogen-bond acceptors so that their FMOs are only slightly affected by hydrogen-bond interactions. Conversely, it is known that nitrones are capable to complex Lewis acids,75-77 thus their FMOs are stabilised in protic solvents. Since nitrone cycloadditions to electron rich ethylenes are usually controlled by the LUMO of the dipole, a better LUMOnitrone-HOMOdipolarophile interaction can be envisaged in water. On the other hand, the main reason that explains the observed rate enhancements are mainly due to the hydrophobic effect.53,54 This statement is substantiated by the beneficial effect of alkali metal salts used as additives, which enhance the ionic strenght of the aqueous medium. A further evidence is provided by the product outcome. Major anti isoxazolidines arise from the corresponding endo transition states which are tighter than the diastereoisomeric exo one48 so that the former can minimise its barely polar surface with respect to bulk water.
An interesting development of the nitrone-olefine cycloaddition in water is related to the presence of a surfactant in the aqueous medium; this protocol was used to synthesise the novel amino acid (24) with orthogonal functionality to the natural amino acid side chains (Scheme 11).78
C,N-Diarylnitrones (25) were generated in situ by the appropriate precursors in the presence of 2-cyclopenten-1-one and sodium dodecylsulfate (SDS) (Scheme 12).78 The SDS concentration was set as 100 mM. It may be useful to recall that: (i) the definition of the critical micellar concentration (CMC) states that "it is the concentration above which molecular aggregates, or micelles, are formed in solution";79 and (ii) the CMC value for SDS is known to be 8.2 mM.8 Thus, the generation of nitrones (25) and the subsequent cycloaddition occur into a micelle being favoured by the high local concentration of the reagents, since the inner part of the micelle behaves as an hydrophobic nanoreactor.
The plot of LogkR/kH as a function of Hammett σp is linear with slope ρ = – 0.94. Such value is consistent with a transition state less polar than the reagents, thus suggesting that the cycloaddition should be faster with electron-donating R.
In water or aqueous medium, the presence of the surfactant plays a key role in the generation step of nitrones. This is apparent by considering the formation extent of N-phenyl-C-(2-nitrophenyl)nitrone (26a) by condensation between phenylhydroxylamine and 2-nitrobenzaldehyde (Table 10).80
Nitrones (26) have been submitted to reaction with ethyl acrylate in the presence of a surfactant giving complex mixtures of regio- and stereoisomeric isoxazolidines (Scheme 13, Table 11).80 The detailed composition of these mixtures was established through NOE experiments.
The effect of a chiral hydrophobic environment onto the nitrone-olefin cycloaddition was studied by using 3,12-diacryloyl sodium deoxycholate (29) as dipolarophilic surfactant (Scheme 14).60 Treatment of (29) with C,N-diphenylnitrone in water gave a mixture of the corresponding regio- and stereoisomeric steroidal isoxazolidines.
It was reported that in water-based microemulsions the ethylenic dipolarophile can act both as a reagent and as a component of the microemulsion.81 The cycloaddition between nitrones (25) and acrylonitrile has been exploited as a function of the water molar fraction in the microemulsion that constitutes the reaction medium. In the case of the microemulsion composed by water (20%), acrylonitrile (50%), n-butanol (20%) and SDS (10%) the formation of an inverse micelle occurs, in which the water droplets are dispersed in acrylonitrile. It was supposed that the nitrone accumulates to the interface between water and acrylonitrile; nitrone reactivity may be spurred as a result of its high local concentration. Compared to the cycloadditions between nitrones (25) and acrylonitrile carried out in refluxing toluene, the reactions in microemulsions were featured with mild conditions (25 °C) and better yields (60-80%), although mixtures of diastereoisomeric isoxazolidines (1) (R = CN) were always obtained.
Cycloaddition between (25) and acrylonitrile has been also pursued in the presence of metal triflates as water-soluble Lewis acid catalysts.81 The effectiveness of these additives was shown to decrease in the following order: Cu(OTf)2 > Sc(OTf)3 > La(OTf)3 > Yb(OTf)3.
II.3. Nitrone-acetylene cycloadditions
The nitrone-alkyne cycloaddition carried out in organic solvents is a well-known reaction.82-84 Surprisingly, very few examples of this process have been described in aqueous medium.
Second-order rate constants were measured for the cycloadditions between nitrones (29) and the highly-strained dibenzocyclooctynol (30) in water/acetonitrile mixtures (Scheme 15, Table 12).85 This methodology was used in a one-pot three-step protocol for the site-selective modification of peptides and proteins. The cycloaddition rate was dependent by the electronic features of R2; electron-withdrawing groups speed up the reaction rate due to the better charge localisation of the dipolar reactant. It needs to be added that the presence of water in the reaction medium did not enhance the reaction rate compared to toluene (see footnote b in Table 12). Dibenzo-azacyclooctyne (32) (Figure 1) was also used as strained dipolarophile, its cycloaddition with N-methyl-C-(benzyl)amidocarbonyl-nitrone was 17 times faster than that with the less polar dibenzocyclooctynol (30) (Figure 1).
III. INTRAMOLECULAR CYCLOADDITIONS
Compared to the corresponding intermolecular process, intramolecular nitrone cycloadditions usually display: (i) better reactivity due to the favourable activation entropies, (ii) better stereoselectivity, and (iii) the opportunity to obtain fused- or bridged isoxazolidines.86
As far as the aqueous medium is concerned, the allyloxynitrone (33) was generated in situ by treating the corresponding phenylhydroxylamine in the presence of a surfactant (Scheme 16, Table 13).87
Reaction times listed in Table 13 are greater than that measured in toluene,88 but in this latter case the reaction was performed at 110 °C. The intramolecular cycloaddition leading to the chromano[4,3-c]- isoxazolidine (34) was also attempted in water/cosolvent mixtures but the results were poor due to the ability of the cosolvent to destroy the micelles (Table 14).
IV. ORGANOCATALYSED CYCLOADDITIONS
The reaction between C-phenyl-N-benzylnitrone and methyl 2-octinoate represents the first example of organocatalysed nitrone cycloaddition in aqueous medium.93 Several molecules with different steric and electronic features were chosen as the organocatalyst (Scheme 19, Table 16). The isoxazoline cycloadduct (37) was obtained with variable yield only in the presence of the organocatalyst, while no reactions occurred in organic solvents. The best results were achieved in the presence of lithium chloride as the additive, in such cases the increase of ionic strenght drives to the enhancement of the hydrophobic effect. The authors recommend to add the reagents to the aqueous medium following the order nitrone-alkynoate (2 equiv.)-catalyst.
IV. ORGANOCATALYSED CYCLOADDITIONS
The reaction between C-phenyl-N-benzylnitrone and methyl 2-octinoate represents the first example of organocatalysed nitrone cycloaddition in aqueous medium.93 Several molecules with different steric and electronic features were chosen as the organocatalyst (Scheme 19, Table 16). The isoxazoline cycloadduct (37) was obtained with variable yield only in the presence of the organocatalyst, while no reactions occurred in organic solvents. The best results were achieved in the presence of lithium chloride as the additive, in such cases the increase of ionic strenght drives to the enhancement of the hydrophobic effect. The authors recommend to add the reagents to the aqueous medium following the order nitrone-alkynoate (2 equiv.)-catalyst.
Recently, an interesting organocatalytic strategy has been developed which allows the one-pot synthesis of tetrahydronaphthyl isoxazolidines in aqueous medium at rt by using 2-(S)-(diphenyltrimethylsilyl)- methylpyrrolidine (39) as the organocatalyst (Jørgensen organocatalyst, Scheme 21, Table 18).94 Fused tricyclic isoxazolidines (40) have been obtained with very high diastereoselectivity; this result is worth of noting because of the five stereocentres of the target molecules, furthermore the enantiomeric excesses were always > 99%.
The catalytic cycle depicted in the Figure 3 give some mechanistic insight about the formation of products (40). The initial role of the organocatalyst (39) is to convert the carbonyl reagent into the corresponding iminium salt. Subsequent tautomerisation give a transient enamine which is able to give a stereoselective Michael addition to the nitrostyrene moiety. Next, the nitrone intermediate (41) is generated and the following stereoselective intramolecular cycloaddition give the target products (40). In summary, this organocatalytic reaction cascade implies the sequence: stereoselective Michael addition/nitrone generation/stereoselective intramolecular nitrone cycloaddition.
The organocatalytic approach based upon stereoselective Michael addition to nitrostyrenes was also effective in the one-pot synthesis of enantiopure nitrones in water, the best organocatalyst for this reaction being the chiral pyrrolidine (39) (Scheme 22, Table 19).95 The γ-nitroaldehyde intermediates were not isolated since their reduction occurred in situ giving enantiopure nitrones (42) with e.e. > 99%.
By using 5-hexenal as the starting aldehyde, the corresponding nitrones were not isolable since their spontaneous stereoselective cycloaddition occurred giving the tricyclic isoxazolidines (43) as single diastereoisomer in the enantiopure form (Scheme 23).95
CONCLUSIONS
The present review describes the development of 1,3-dipolar cycloadditions of nitrones in water or aqueous media. Examples of intermolecular as well as intramolecular cycloadditions were discussed focusing on the advantages with respect to the cycloadditions performed in organic solvents. The interplay of factors driving to the rate acceleration of the 1,3-dipolar cycloadditions in aqueous media were discussed giving a picture of the beneficial hydrophobic effect at work in the 1,3-dipolar cycloadditions of nitrones. The valuable effects produced by surfactants and the fruitful water-based organocatalytic approach of nitrone cycloadditions were also described.
References
1. R. P. Schwarzenbach, P. M. Gschwend, and D. M. Imboden, 'Environmental Organic Chemistry', John Wiley & Sons, Inc., New York, 1993, pp. 157-181.
2. M. C. Pirrung and K. Das Sarma, J. Am. Chem. Soc., 2004, 126, 444. CrossRef
3. R. Breslow and D. C. Rideout, J. Am. Chem. Soc., 1980, 102, 7816. CrossRef
4. W. Blokzijl, M. J. Blandamer, and J. B. F. N. Engberts, J. Am. Chem. Soc., 1991, 113, 4241. CrossRef
5. S. Otto and J. B. F. N. Engberts, Pure Appl. Chem., 2000, 72, 1365. CrossRef
6. J. W. Wijnen, R. A. Steiner, and J. B. F. N. Engberts, Tetrahedron Lett., 1995, 36, 5389. CrossRef
7. R. N. Butler, A. G. Coyne, W. J. Cunningham, and L. A. Burke, J. Chem. Soc., Perkin Trans. 2, 2002, 1807. CrossRef
8. D. van Mersbergen, J. W. Wijnen, and J. B. F. N. Engberts, J. Org. Chem., 1998, 63, 880. CrossRef
9. J. Dambacher, W. Zhao, A. El-Batta, R. Anness, C. Jiang, and M. Bergdahl, Tetrahedron Lett., 2005, 46, 4473. CrossRef
10. K. Kacprzak, Synlett, 2005, 943. CrossRef
11. S. Narayan, J. Muldoon, M. G. Finn, V. V. Fokin, H. C. Kolb, and K. B. Sharpless, Angew. Chem. Int. Ed., 2005, 44, 3275. CrossRef
12. K. Kranjc, M. Kočevar, F. Iosif, S. M. Coman, V. I. Parvulescu, E. Genin, J.-P. Genêt, and V. Michelet, Synlett, 2006, 1075. CrossRef
13. N. Chatterjee, P. Pandit, S. Halder, A. Patra, and D. K. Maiti, J. Org. Chem., 2008, 73, 7775. CrossRef
14. C.-J. Li, Chem. Rev., 1993, 93, 2023. CrossRef
15. C.-J. Li, Chem. Rev., 2005, 105, 3095. CrossRef
16. C.-J. Li and L. Chen, Chem. Soc. Rev., 2006, 35, 68. CrossRef
17. U. M. Lindström, Chem. Rev., 2002, 102, 2751. CrossRef
18. A. Chanda and V. V. Fokin, Chem. Rev., 2009, 109, 725. CrossRef
19. R. N. Butler and A. G. Coyne, Chem. Rev., 2010, 110, 6302. CrossRef
20. J. Hamer and A. Macaluso, Chem. Rev., 1964, 64, 473. CrossRef
21. W.-M. Shi, X.-P. Ma, G.-F. Su, and D.-L. Mo, Org. Chem. Front., 2016, 3, 116. CrossRef
22. L. L. Anderson, Asian J. Org. Chem., 2016, 5, 9. CrossRef
23. C.-J. Li and T.-H. Chan, 'Organic Reactions in Aqueous Media,' Wiley, New York, 1997.
24. 'Organic Reactions in Water: Principles, Strategies and Applications,' ed. by U. M. Lindström, Blackwell Publishing, Oxford, 2007.
25. 'Organic Reactions in Water: Principles, Strategies and Applications,' ed. by U. M. Lindström, Blackwell Publishing, Oxford, 2007.
26. 'Water in Organic Synthesis', ed. by S. Kobayashi, Georg Thieme Verlag, Stuttgart, 2012.
27. S. Moulay and A. Touati, Compt. Rend. Chim., 2010, 13, 1474. CrossRef
28. G. Molteni, Heterocycles, 2006, 68, 2177. CrossRef
29. G. Molteni, 'Water in Organic Synthesis,' ed. by S. Kobayashi, Georg Thieme Verlag, Stuttgart, 2012, pp. 433-479.
30. L. Zengmin, S. Tae Seok, and J. Jingyue, Tetrahedron Lett., 2004, 45, 3143. CrossRef
31. Z.-X. Wang and H.-L. Qin, Chem. Commun., 2003, 2450. CrossRef
32. G. Molteni and A. Ponti, ARKIVOK, 2006, xvi, 49.
33. A. A. Ali, M. Chetia, and D. Sarma, Tetrahedron Lett., 2016, 57, 1711. CrossRef
34. P. Del Buttero and G. Molteni, Tetrahedron, 2011, 67, 7343. CrossRef
35. C. Kesornpun, T. Aree, C. Mahidol, S. Ruchirawat, and P. Kittakoop, Angew. Chem. Int. Ed., 2005, 44, 3997.
36. G. Molteni, A. Ponti, and M. Orlandi, New J. Chem., 2002, 26, 1340. CrossRef
37. A. Ponti and G. Molteni, New J. Chem., 2002, 26, 1346. CrossRef
38. G. Molteni, Tetrahedron: Asymmetry, 2004, 15, 1077. CrossRef
39. G. Molteni and P. Del Buttero, Heterocycles, 2005, 65, 1183. CrossRef
40. S. Dadiboyena and A. T. Hamme II, Eur. J. Org. Chem., 2013, 7567. CrossRef
41. R. C. F. Jones, 'Synthetic Applications of 1,3-Dipolar Cycloaddition Chemistry Toward Heterocycles and Natural Products', ed. by A. Padwa, John Wiley & Sons, Inc., New York, 2002, pp. 1-83. CrossRef
42. G. La Sorella, G. Strukul, and A. Scarso, Green Chem., 2015, 17, 644. CrossRef
43. E. Beckmann, Ber. Dtsch. Chem. Ges., 1890, 2, 565.
44. L. I. Smith, Chem. Rev., 1938, 23, 193. CrossRef
45. R. Huisgen, Angew. Chem., Int. Ed. Engl., 1963, 2, 565. CrossRef
46. R. Huisgen, Angew. Chem., Int. Ed. Engl., 1963, 2, 633. CrossRef
47. R. Huisgen, '1,3-Dipolar Cycloaddition Chemistry', ed. by A. Padwa, Wiley, New York, 1984, Vol. 1, pp. 1-176.
48. P. N. Confalone and E. M. Huie, Org. React., 1988, 36, 1.
49. J. J. Tufariello, '1,3-Dipolar Cycloaddition Chemistry', ed. by A. Padwa, Wiley, New York, 1984, Vol. 1, pp. 83-168.
50. 'Nitrile Oxides, Nitrones, and Nitronates in Organic Synthesis', ed. by H. Feuer, John Wiley & Sons, Inc., New York, 2008.
51. K. N. Houk, J. Sims, R. E. Duke, R. W. Strozier, and J. K. George, J. Am. Chem. Soc., 1973, 95, 7287. CrossRef
52. K. N. Houk, J. Sims, C. R. Watts, and L. J. Luskus, J. Am. Chem. Soc., 1973, 95, 7301. CrossRef
53. N. T. Southall, K. A. Dill, and A. D. J. Haymet, J. Phys. Chem. B, 2002, 106, 521. CrossRef
54. W. Blokzijl and J. B. F. N. Engberts, Angew. Chem., Int. Ed. Engl., 1993, 32, 1545. CrossRef
55. T. Asano and H. Le Noble, Chem. Rev., 1978, 78, 407. CrossRef
56. R. van Eldik, T. Asano, and W. J. Le Noble, Chem. Rev., 1989, 89, 549. CrossRef
57. M. R. Gholami and A. H. Yangjeh, J. Chem. Res., Synop., 1999, 226. CrossRef
58. C. Reichardt, 'Solvents and Solvent Effects in Organic Chemistry', Wiley-VCH Verlag GmbH & Co., Weinheim, 2003, pp. 389-470.
59. M. R. Gholami and A. H. Yangjeh, Int. J. Chem. Kinet., 2000, 32, 431. CrossRef
60. P. S. Pandey and I. K. Pandey, Tetrahedron Lett., 1997, 38, 7237. CrossRef
61. R. Gandolfi and P. Grünanger, Chem. Heterocycl. Compd., 1999, 49, 774.
62. E. Coutolis-Argyropoulou, P. Sarridis, and P. Ckizis, Green Chem., 2009, 11, 1906. CrossRef
63. O. M. Musa and L. M. Sridhar 2009, Patent WO2009136920 A1.
64. B. Chakraborty, P. Sharma, S. Kafley, M. S. Chetri, and A. R. Ghosh, Rasayan J. Chem., 2009, 2, 946.
65. B. Chakraborty, P. K. Sharma, and M. S. Chetri, J. Heterocycl. Chem., 2012, 49, 1260. CrossRef
66. B. Chakraborty, A. Samanta, P. K. Sharma, M. S. Chhetri, S. Kafley, A. Banerjee, and C. Sinha, J. Chem. Pharm. Res., 2010, 2, 727.
67. G. Singh, A. Sharma, H. Kaur, and M. P. Ishar, Chem. Biol. Drug Des., 2016, 87, 213. CrossRef
68. B. Chakraborty, M. S. Chetra, and A. Samanta, Indian J. Chem., 2010, 49 B, 1155.
69. E. Coutolis-Argyropoulou, P. Sarridis, and P. Ckizis, Green Chem., 2009, 11, 1906. CrossRef
70. V. Liautard, V. Desvergnes, and O. R. Martin, Tetrahedron: Asymmetry, 2008, 19, 1999. CrossRef
71. M. Greittner, R. Huisgen, and R. Reissig, Heterocycles, 1978, 18, 109.
72. R. Huisgen, M. Seidl, and H. Brüning, Chem. Ber., 1969, 102, 1102. CrossRef
73. A. Lattes, I. Rico, A. de Savignac, and A. A. Samii, Tetrahedron, 1987, 43, 1725. CrossRef
74. I. Fleming, 'Molecular Orbitals and Organic Chemical Reactions, Reference Edition', John Wiley & Sons, Ltd., Chichester, 2010, pp. 253-368. CrossRef
75. F. A. Villamena, M. H. Dickman, and L. R. DeCrist, Inorg. Chem., 1998; 37, 1446. CrossRef
76. J. Lee, B. Twamley, and G. B. Richter-Addo, J. Chem. Soc., Chem. Commun., 2002, 380. CrossRef
77. P. Das, M. Boruayh, N. Kumari, M. Sharma, D. Konwar, and D. K. Dutta, J. Mol. Catal. A, 2002, 178, 283. CrossRef
78. G. R. Lorello, M. C. B. Legault, B. Rakic, K. Bisgaard, and J. P. Pezacki, Bioorg. Chem., 2008, 36, 105. CrossRef
79. D. J. Shaw, 'Introduction to Colloid and Surface Chemistry', Butterworth, Oxford, 1992, pp. 64-114. CrossRef
80. A. Chatterjee, D. K. Maiti, and P. K. Bhattacharya, Org. Lett., 2003, 5, 3967. CrossRef
81. K. Hamza and A. Touati, Asian J. Chem., 2010, 22, 1231.
82. J. Bastide and O. Henri-Rousseau, 'The Chemistry of the Carbon-carbon Triple Bond', ed. by S. Patai, John Wiley & Sons., Inc., New York, 1978, Vol. 1, pp. 447–522. CrossRef
83. K. N. Houk, Heterocycles, 1977, 7, 293. CrossRef
84. R. Huisgen, H. Seidl, and I. Brüning, Chem. Ber., 1969, 102, 1102. CrossRef
85. X. Ning and R. P. Temming, Angew. Chem. Int. Ed., 2010, 49, 3065. CrossRef
86. P. A. Wade, 'Comprehensive Organic Synthesis', ed. by B. M. Trost, I. Fleming, and M. F. Semmelhack, Pergamon Press, Oxford, 1991, Vol. 4, pp. 1111–1168. CrossRef
87. A. Chatterjee, S. K. Hota, M. Banerjee, and P. K. Bhattacharya, Tetrahedron Lett., 2010, 51, 6700. CrossRef
88. W. Oppolzer and H. P. Weber, Tetrahedron Lett., 1970, 1117. CrossRef
89. A. Chatterjee and P. K. Bhattacharya, J. Org. Chem., 2006, 71, 345. CrossRef
90. M. Baunach and C. Hertweck, Angew. Chem. Int. Ed., 2015, 54, 12550. CrossRef
91. X.-J. Wang, G.-J. Zhang, P.-Y. Zhuang, Y. Zhang, S.-S. Yu, X.-Q. Bao, D. Zhang, Y.-H. Yuan, N.-H. Chen, S.-G. Ma, J. Qu, and Y. Li, Org. Lett., 2012, 14, 2614. CrossRef
92. E. H. Krenske, A. Patel, and K. N. Houk, J. Am. Chem. Soc., 2013, 135, 17638. CrossRef
93. D. G. Cruz, D. Tejedor, P. de Armas, E. Q. Morales, and F. G. Tellado, Chem. Commun., 2006, 2798. CrossRef
94. B. Tan, D. Zhu, L. Zhang, P. J. Chua, X. Zeng, and G. Zhong, Chem. Eur. J., 2010, 16, 3842.. CrossRef
95. D. Sádaba, I. Delso, T. Tejero, and P. Merino, Tetrahedron Lett., 2011, 52, 5976. CrossRef