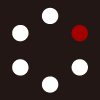
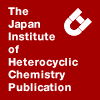
HETEROCYCLES
An International Journal for Reviews and Communications in Heterocyclic ChemistryWeb Edition ISSN: 1881-0942
Published online by The Japan Institute of Heterocyclic Chemistry
e-Journal
Full Text HTML
Received, 25th August, 2016, Accepted, 21st October, 2016, Published online, 7th November, 2016.
■ Synthesis of Quinoline Derivatives by an Improved Döebner-von Miller Reaction Using a Recyclable Ag(I)-Exchanged Montmorillonite K10 Catalyst
Janeeka Jayram and Vineet Jeena*
School of Chemistry and Physics, University of KwaZulu-Natal, Cnr Golf and Ridge Roads 3209, South Africa
Abstract
The Döebner-von Miller synthesis of quinolines under solvent-free conditions is described, catalyzed by recyclable Ag(I)-exchanged Montmorillonite K10. The desired products are obtained in good to excellent yields and the reaction accommodates both aromatic and aliphatic α,β-unsaturated aldehydes making this process an attractive method for the synthesis of substituted quinolines. In addition, the Ag(I)-exchanged Montmorillonite K10 catalyst can be recovered and reused up to 5 times with excellent activity.In 1881, German scientists Oscar Döebner and Wilhelm von Miller reported the synthesis of quinolines from aniline and α,β–unsaturated carbonyl compounds.1 This reaction subsequently named the Döebner-von Miller reaction has attracted great attention owing to its operational simplicity2 and easy formation of quinoline derivatives from inexpensive starting materials. The reaction, though, is characterized by harsh conditions and a tedious isolation procedure from complex reaction mixtures.3 In addition, the yields are relatively low, owing to many by-products due to polymerization of the α, β-unsaturated aldehyde.4 Consequently, studies on the mechanism of the Döebner-von Miller reaction has long been a subject of interest.5
Given the biological and pharmacological importance of quinoline derivatives,6 the development of a simple, high-yielding and environmentally friendly approach towards the synthesis of these compounds, using the Döebner-von Miller reaction, is of utmost importance. Numerous efforts have thus been devoted to finding an advanced method to further improve this reaction in order to overcome the numerous challenges stated above. One such example is the development of a “two-phase” Döebner-von Miller reaction, which decreases the polymerization of the aldehyde, producing the desired product in moderate yields using hydrochloric acid (HCl) as a catalyst (Scheme 1).7a,b Another example is the use of the diethyl acetal of acrolein as an annulation partner in the synthesis of quinoline products (Scheme 1).7c
In an effort to further develop the Döebner-von Miller reaction, our attention became focused on the use of clays as a catalyst towards quinoline synthesis. The application of natural and modified clays as solid acid catalysts for chemical reactions constitutes an exciting component of green chemistry.8 Various heterocyclic syntheses involve the common solid acid catalyst, Montmorillonite K10 (Mont-K10), producing diarylpyrazoles,9a pyridines,9b pyrazines9b and pyrimidines.9b Montmorillonite K10, Al2Si4O10(OH)2.nH2O, is a negatively charged silicate with excellent cation exchange properties10 allowing alteration of the acidic sites by a simple ion-exchange procedure.11
Cationic-exchanged catalysts have been well documented as solid-acid catalysts for various organic transformations12 and Ag(I)-exchanged Montmorillonite K10 (Ag(I)-Mont-K10), in particular, has been shown to display excellent activity in the synthesis of propargylamines13 and substituted isoquinolines.14 Metal-exchanged clays possess catalytic abilities in their own right as an acid or redox catalyst without any additional treatment15 and the acid strength of Montmorillonite K10 increases with decreasing amounts of the water interlayer and is affected by the position of the negative charge of the clay.16 An increase in the dissociation of the water of hydration causes stronger and more numerous acid sites available for cation exchange. Therefore, the catalytic activity arises from the intrinsic activity of silver ions and surface acidity due to cation exchange.16 Hence, the addition of the silver ions onto the surface of the clay decreases the water interlayer consequently increasing the acidity of the catalyst resulting in higher yields compared to natural Montmorillonite K10.13
Given the unsatisfactory yields in the Döebner-von Miller reaction, we were interested in exploiting the potential application of Ag(I)-exchanged Montmorillonite K10 in the synthesis of substituted quinolines. We envisioned that Ag(I)-exchanged Montmorillonite K10 would be a suitable solid support and sufficiently acidic to promote cyclization and minimize polymerization of the α,β-unsaturated aldehyde. We describe herein, a mild and efficient synthesis of quinoline derivatives via the Döebner-von Miller reaction, using Ag(I)-exchanged Montmorillonite K10, as a recyclable, non-toxic catalyst as an alternate approach (Scheme 2) to previously described methods (vide supra).
The silver-modified clay was prepared by an ion-exchanged method and characterized by Scanning Electron Microscopy (SEM) and Energy Dispersion X-ray (EDX) analysis. Comparison of the SEM images showed a distinct change in the surface morphology brought about by the addition of silver ions (see ESI, S2) and the total silver content was found to be 3.67 weight % by EDX analysis (See ESI, S5). Montmorillonite K10 and Ag(I)-exchanged Montmorillonite K10 were then used to optimize reaction conditions as part of a comparison study since natural Montmorillonite K10 has been shown to display promising results in the Döebner-von Miller reaction.17
The study commenced by probing a test reaction between excess aniline and cinnamaldehyde under various reaction conditions and the results are illustrated in Table 1 as the use of excess aniline has been shown to have a pronounced effect on the reaction yield.17 We began our studies under various sonication conditions and in all cases; the yield of the desired product was disappointing (Table 1, entries 1–4). We then turned our attention to conventional heating conditions in the presence of toluene, and the desired product was obtained in moderate yields (Table 1, entries 5-6). Reactions were subsequently performed under solvent-free conditions resulting in a notable increase in the yield of the product (Table 1, entries 7-8), particularly in the case of Ag(I)-exchanged Montmorillonite K10. Thus, the reaction was repeated for 3 hours and, to our delight, the desired quinoline product was obtained in a yield of 89% (Table 1, entry 9). It is interesting to note that the present experimental condition resulted exclusively in the formation of the desired product, 2-phenylquinoline, using Ag(I)-Mont-K10 clay as a catalyst, and no other byproducts were observed. When the reaction was performed for longer than 3 hours, trace byproducts were detected. A blank control experiment was conducted and, in this case, no product was observed (Table 1, entry 10) indicating the need for a solid acid catalyst. It is also worth mentioning that Ag(I)-exchanged Montmorillonite K10 was found to be superior to natural Montmorillonite K10 in all examples.
With the optimized conditions in hand, we surveyed the functional group compatibility and explored the scope of this system by using a series of substituted anilines with cinnamaldehyde (2a) and the results are summarized in Table 2. As the data indicates, all substituted anilines gave moderate to excellent yields (Table 2, 3b-f). In the case of meta-substituted aniline (Table 2, 3f) a regioselective para cyclization occurred, preventing the formation of an isomeric mixture thus yielding 7-methyl-2-phenylquinoline exclusively with unreacted starting material recovered. It is interesting to note that anilines with strong electron-withdrawing groups (nitroaniline) showed diminished reactivity toward quinoline synthesis, which had a negative effect on yields (trace) (Table 2, 3g and 3h). These results are consistent with other examples involving the Döebner-von Miller reaction using nitroaniline.7b,17 We then commenced with the variation of the aldehyde and the system was equally efficient (Table 2, 3i). In an attempt to further widen the applicability of our system, we selected the reaction between 6-chloro-/6-bromo-aniline and crotonaldehyde. In these cases, crotonaldehyde readily underwent cyclization to form quinolines in excellent yields (Table 2, 3j and 3k). It is particularly gratifying that a variety of functional groups, such as methyl, ethyl, chloro, bromo and methoxy were well tolerated in the present protocol and, in all cases, minimal by-product formation was observed.
Numerous examples in literature deal exclusively with either aromatic α, β-unsaturated aldehydes17 or aliphatic α,β-unsaturated aldehydes.2 To the best of our knowledge, studies of the Döebner-von Miller reaction that is equally efficient on both aromatic and aliphatic α, β-unsaturated aldehydes has not been reported. A literature review comparison study was conducted to evaluate our system (see ESI, S6) and in most cases, a vast improvement in yield, than those reported in literature, was obtained highlighting the scope of our method to both aromatic and aliphatic α,β-unsaturated aldehydes. In addition, the Ag(I)-exchanged Montmorillonite K10 approach is milder than previously reported syntheses.
Despite the numerous instances of successful quinoline syntheses, the mechanism of the Döebner-von Miller reaction has long been a subject of debate and is quite controversial,5,18-20 therefore, the Ag(I)-exchanged Montmorillonite K10 mediated approach is speculated to follow the path described by Török and co-worker.17 Initially, aniline adds to the α, β-unsaturated aldehyde resulting in the formation of the imine, catalyzed by Ag(I)-exchanged Montmorillonite K10. It is expected that the catalyst plays a role in this step as it has been reported that imine formation is favored by the presence of a clay catalyst.21 The imine is then attacked by a second molecule of aniline resulting in the formation of an intermediate, followed by the removal of aniline to allow cyclization yielding a second intermediate, which finally affords the desired quinoline.
In order to further support the argument that the second molecule of aniline is essential, we performed reactions using equimolar quantities of aniline and cinnamaldehyde, in the presence of natural Montmorillonite K10 and Ag(I)-exchanged Montmorillonite K10, using the optimized reaction conditions (Table 1, entry 9) and the results are illustrated in Table 3.
In both cases, diminished yields of the quinoline product were obtained when 1 equivalent of aniline was used, justifying the need for excess nucleophile. In view of the above-proposed mechanism and extensive literature research dating back to 1881, the results of this study lend considerable support to the mechanism proposed by Tung in 1963, who claimed that the first step of the Döebner von-Miller reaction is imine formation and that excess aniline is required for a successful quinoline synthesis.22
The possibility of recycling the catalyst by simple filtration, without the loss of activity is one of the key advantages of heterogeneous catalysis over the homogeneous counterpart.23 Thus, the recovery and reusability of the catalyst were investigated in the quinoline formation using the optimized test reaction (Table 1, entry 9). After completion of the reaction, the catalyst was recovered by simple filtration and dried. The recovered catalyst was weighed after each run and its activity examined using the test reaction. In order to gain an accurate assessment of the activity of the catalyst, each run was repeated using fresh Ag(I)-exchanged Montmorillonite K10 (Figure 1). We observed that the yields of the product remained comparable irrespective of whether recovered or fresh Ag(I)-exchanged Montmorillonite K10 was used. These results also indicated that the catalyst loading of 0.5 g was critical for a successful reaction as lower catalyst loadings resulted in diminished yields (0.5 g, 89% vs 0.43 g, 74%).
In summary, we have developed a simple and convenient Ag(I)-exchanged Montmorillonite K10 catalyzed synthetic route for the formation of substituted quinoline derivatives from aromatic amines and α,β-unsaturated aldehydes. A series of substituted quinoline derivatives were synthesized in good to excellent yields from commercially available and inexpensive starting materials via the Döebner-von Miller reaction. In addition, the derived synthetic procedure accommodates both aromatic and aliphatic α, β-unsaturated aldehydes. The solid catalyst can be readily recovered by simple filtration and reused several times without any appreciable loss in activity, thus making this procedure more environmentally benign. The mechanism is speculated to proceed by the formation of the imine, addition of a second molecule of aniline followed by subsequent cyclization and aromatization yielding the desired quinoline. Current efforts are underway to further expand the scope and limitations of this approach to complex substrates as well as further elucidating the mechanism of the Döebner-von Miller reaction.
EXPERIMENTAL
All starting reagents were purchased from Sigma-Aldrich and used without further purification. Nuclear Magnetic Resonance (NMR) spectra were recorded on Bruker Advance 400 III operating at either 400 or 100 MHz. All 1H and 13C NMR spectra were measured in CDCl3. Chemical shifts are expressed in parts per million (ppm) relative to the residual solvent and coupling constants (J) were recorded in Hz. NMR spectra were referenced against the residual CDCl3 present in δH 7.26 ppm and δC 77.0 ppm. Chromatograms were obtained using Perkin Elmer Clarus 500 Gas Chromatograph. IR spectra were recorded on Smiths IdentifyIR® Spectrometer. Melting points were determined using Kofler hot-stage melting apparatus and are uncorrected. Purifications by column chromatography were performed on silica gel 40 - 60 mesh. The morphology of Montmorillonite K10 and Ag(I)-exchanged Montmorillonite K10 particles was determined by Scanning Electron Microscopy (SEM) (EVO LS15, ZEISS Company, Germany). The Energy Dispersion X-ray (EDX) analysis was performed with an X-max 80 mm2 Silicone Drift Detector (SDD), coupled with the SEM to analyze the elemental composition of the samples. All synthesized compounds are known and their spectroscopic data in agreement with literature with the appropriate references being provided.
General procedure for the synthesis of quinoline derivatives
Amine (1.50 mmol) and aldehyde (1.00 mmol) were dissolved in 1.5 mL of Et2O in a reaction vial equipped with a magnetic stirrer bar, followed by the addition of Ag(I)-exchanged Montmorillonite K10 (0.50 g). After 5 min stirring, the solvent was removed in vacuo to obtain a dry powder. The reaction mixture was heated at a temperature of 120 oC for 3 h. After cooling to room temperature, the reaction mixture was filtered through a short silica plug and the solid residues washed well with CH2Cl2. The solvent was removed in vacuo and the crude product purified by column chromatography over silica gel eluting with a mixture of Hexane : EtOAc to produce the title compounds (3a-3k). NOTE. For the recycle/reuse study, the crude product was gravity filtered and the clay material washed several times with CH2Cl2, air dried and weighed. This process was repeated for consecutive recycle/reuse reactions.
2-Phenylquinoline (Table 2, 3a): yellow solid: (0.183 g, 89%); (mp 82-84 °C) (lit 84-85 °C); Rf 0.67 (20 : 1 Hexane : EtOAc); 1H NMR (400 MHz, CDCl3) δH 7.46-7.51 (1H, m), 7.53-7.56 (3H, m), 7.73-7.77 (1H, m), 7.85 (1H, d, J = 8.31 Hz), 7.88-7.91 (1H, d, J = 8.31 Hz), 8.18-8.27 (4H, m); 13C NMR (100 MHz) δC 119.2, 126.7, 127.2, 127.5, 127.9, 128.4, 128.7, 128.9, 129.8, 130.3, 137.9, 157.2; vmax (neat, cm-1): 3033, 2970, 1489, 1319, 1284 cm−1; MS (m/z, %): 206 (MH+) (15), 205 (100), 204 (92), 203 (12). Data consistent with literature.24
6-Chloro-2-phenylquinoline (Table 2, 3b): white solid: (0.135 g, 56%); (mp 108-111 oC) (lit 108-110 °C); Rf 0.38 (20 : 1 Hexane : EtOAc); 1H NMR (400 MHz, CDCl3) δH 7.48 (1H, t, J = 7.0 Hz), 7.54 (2H, t, J = 7.5 Hz), 7.67 (1H, dd, J = 8.9, 2.1 Hz), 7.82 (1H, d, J = 2.6 Hz), 7.92 (1H, d, J = 9.4 Hz ), 8.15 (2H, m, J = 8.0 Hz), 8.15-8.16 (1H, m), 8.16-8.17 (1H, m); 13C NMR (100 MHz) δC 119.9, 126.4, 127.6, 127.8, 128.9, 129.7, 130.7, 131.4, 132.0, 136.0, 139.3, 146.8, 157.6; vmax (neat, cm-1): 3055, 2918, 1464, 1330, 831 cm−1; MS (m/z, %): 240 (MH)+ (28), 239(100), 238 (41). Data consistent with literature.25,26
6-Bromo-2-phenylquinoline (Table 2, 3c): white solid: (0.121 g, 42%); (mp 111-113 °C) (lit 112-113 °C); Rf 0.54 (20 : 1 Hexane : EtOAc); 1H NMR (400 MHz, CDCl3) δH 7.46-7.56 (3H, m), 7.78 (1H, dd, J = 8.5, 2.2 Hz), 7.91 (1H, d, J = 9.0 Hz), 8.00 (1H, d, J = 2.4 Hz), 8.06-8.18 (4H, m); 13C NMR (100 MHz) δC 119.8, 120.1, 127.5, 128.3, 128.9, 129.5, 129.6, 131.4, 133.1, 135.8, 139.1, 146.8, 157.7; vmax (neat, cm-1): 3055, 2916, 2849, 1459, 1330, 693 cm−1; MS (m/z, %): 284 (MH)+ (48), 283 (100), 282 (35). Data consistent with literature.27, 28
6-Methoxy-2-phenylquinoline (Table 2, 3d): white solid: (0.109 g, 46%); (mp 127-130 oC) (lit 129-130 °C); Rf 0.15 (20 : 1 Hexane : EtOAc); 1H NMR (400 MHz, CDCl3) δH 3.96 (3H, s), 7.11 (1H, d, J = 2.7 Hz), 7.40 (1H, dd, J = 9.2, 3.1 Hz), 7.43-7.54 (3H, m), 7.84 (1H, d, J = 8.7), 8.13-8.15 (4H, m); 13C NMR (100 MHz) δC 55.6, 105.0, 119.4, 122.6, 127.4, 128.2, 128.9, 129.2, 130.8, 135.9, 139.8, 144.6, 154.9, 157.9; vmax (neat, cm-1): 3059, 2899, 1619, 1595, 1489, 1453, 1338 cm−1; MS (m/z, %): 235 (M+) (100), 220 (29), 192 (42). Data consistent with literature.29,30
6-Ethyl-2-phenylquinoline (Table 2, 3e): yellow oil: (0.193 g, 82%); Rf 0.34 (20 : 1 Hexane : EtOAc); 1H NMR (400 MHz, CDCl3) δH 1.36 (3H, t, J = 6.9 Hz), 2.86 (2H, q, J = 7.7 Hz), 7.50 (3H, m), 7.6 (2H, m), 7.85 (1H, d, J = 8.4 Hz), 8.17 (4H, m); 13C NMR (100 MHz) δC 15.4, 28.9, 118.9, 124.9, 127.3, 127.6, 128.8, 129.2, 129.5, 130.9, 136.4, 139.7, 142.5, 146.9, 156.5; vmax (neat, cm-1): 3056, 2856, 1301, 1049, cm−1; MS (m/z, %): 233 (M+) (70), 204 (13), 218 (100). Data consistent with literature.17
7-Methyl-2-phenylquinoline (Table 2, 3f): pale yellow solid: (0.185 g, 84%); (mp 90-93 oC) (lit 92-93 °C); Rf 0.45 (20 : 1 Hexane : EtOAc); 1H NMR (400 MHz, CDCl3) δH 2.59 (3H, s), 7.36 (1H, dd, J = 8.4 Hz, 1.2 Hz), 7.44-7.48 (1H, m), 7.51-7.55 (2H, m), 7.72 (1H, d, J = 8.0 Hz), 7.81 (1H, d, J = 8.8 Hz), 7.99 (1H, s), 8.15-8.18 (3H, m); 13C NMR (100 MHz) δC 21.9, 118.3, 125.3, 127.1, 127.6, 128.5, 128.6, 128.8, 129.3, 136.7, 139.7, 140.1, 148.4, 157.3; vmax (neat, cm-1): 3065, 2873, 1631, 1459 cm−1; MS (m/z, %): 219 (M+) (100), 218 (42), 204 (22). Data consistent with literature.31,32
2-(4-Methoxyphenyl)quinoline (Table 2, 3i): white solid: (0.141 g, 60%); (mp 120-122 oC) (lit 119-121 °C); Rf 0.43 (20 : 1 Hexane : EtOAc); 1H NMR (400 MHz, CDCl3) δH 3.91 (3H, s), 7.06 (2H, d, J = 9.6 Hz), 7.52 (1H, t, J = 7.9 Hz), 7.74 (1H, t, J = 7.6 Hz), 7.82-7.87 (2H, m), 8.16-8.24 (4H, m); 13C NMR (100 MHz) δC 55.4, 114.4, 118.7, 126.3, 126.9, 127.5, 128.5, 129.4, 130.2, 135.6, 137.7, 147.0, 156.5, 161.3; vmax (neat, cm-1): 3061, 2839, 1276, 1062, 1459 cm−1; MS (m/z, %): 236 (MH+) (56), 235 (100), 220 (73). Data consistent with literature.26,33
6-Chloro-2-methylquinoline (Table 2, 3j): yellow solid: (0.143 g, 80%); (mp 95-98 ̊C) (lit 94-98 °C); Rf 0.21 (20 : 1 Hexane : EtOAc); 1H NMR (400 MHz, CDCl3) δH 2.76 (3H, s), 7.32 (1H, d, J = 8.3 Hz), 7.61-7.64 (1H, m), 7.77 (1H, d, J = 8.8), 7.98 (1H, d, J = 3.4 Hz), 8.00 (1H, d, J = 8.9 Hz); 13C NMR (100 MHz) δC 25.1, 122.9, 126.2, 127.0, 129.9, 130.5, 131.6, 135.6, 145.7, 159.2; vmax (neat, cm-1): 3034, 2918, 1487, 1304, 831 cm−1; MS (m/z, %): 178 (MH+) (15), 177 (100). Data consistent with literature.34
6-Bromo-2-methylquinoline (Table 2, 3k): white solid: (0.181 g, 81%); (mp 103-105 oC) (lit 102-106 °C); Rf 0.33 (20 : 1 Hexane : EtOAc); 1H NMR (400 MHz, CDCl3) δH 2.75 (3H, s), 7.32 (1H, d, J = 8.3 Hz), 7.74-7.77 (1H, m), 7.92-7.99 (3H, m); 13C NMR (100 MHz) δC 25.1, 119.6, 122.9, 127.6, 129.5, 130.0, 133.1, 135.6, 145.9, 159.4; vmax (neat, cm-1): 3070, 2914, 1226, 1334, 1487, 1276, 697 cm−1;
MS (m/z): 222 (MH+) (13), 221 (100). Data consistent with literature.34
SUPPLEMENTARY INFORMATION
SEM, EDX and Elemental analysis of Montmorillonite K10 and Ag(I)-exchanged Montmorillonite K10 can be accessed in the “Supplementary Information” section of this article’s webpage.
ACKNOWLEDGEMENTS
We are grateful to the National Research Foundation (NRF) of South Africa for a postgraduate bursary (JJ) and the University of KwaZulu-Natal for financial assistance.
Subashen Naidu (Microscopy and Microanalysis unit, UKZN) is gratefully acknowledged for assisting with the SEM/EDX spectra.
References
1. (a) O. Döebner and W. von Miller, Ber., 1881, 14, 2812; CrossRef (b) O. Döebner and W. von Miller, Ber., 1883, 16, 1664; CrossRef (c) O. Döebner and W. von Miller, Ber., 1883, 16, 246; (d) O. Döebner and W. von Miller, Ber., 1884, 17, 1712. CrossRef
2. G. Sivaprasad, R. Rajesh, and P. T. Perumal, Tetrahedron Lett., 2006, 47, 1783. CrossRef
3. C. M. Leir, J. Org. Chem., 1977, 42, 911. CrossRef
4. S. A. Yamashkin and E. A. Oreshkina, Chem. Heterocycl. Compd., 2006, 42, 701. CrossRef
5. (a) J. J. Eisch and T. Dluzniewski, J. Org. Chem., 1989, 54, 1269; CrossRef (b) S. E. Denmark and S. Venkatraman, J. Org. Chem., 2006, 71, 1668. CrossRef
6. (a) B. Kalluraya and S. Sreenivasa, Il Farmaco, 1998, 53, 399; CrossRef (b) T. Stringer, D. Taylor, H. Guzgay, A. Shokar, A. Au, P. J. Smith, D. T. Hendricks, K. M. Land, T. J. Egan, and G. S. Smith, Dalton Trans., 2015, 44, 14906. CrossRef
7. (a) M. Matsugi, F. Tabusa, and J.–i. Minamikawa, Tetrahedron Lett., 2000, 41, 8523; CrossRef (b) K. A. Reynolds, D. J. Young, and W. A. Loughlin, Synthesis, 2010, 3645; CrossRef (c) G. A. Ramann and B. J. Cowen, Tetrahedron Lett., 2015, 56, 6436. CrossRef
8. R. S. Varma, Tetrahedron., 2002, 58, 1235. CrossRef
9. (a) F. Hatamjafari, Asian J. Chem., 2013, 25, 2339; (b) R. S. Varma and D. Kumar, Tetrahedron Lett., 1999, 40, 7665. CrossRef
10. (a) P. Laszlo, Acc. Chem. Res., 1986, 19, 121; CrossRef (b) J. M. Adams, Appl. Clay Sci., 1987, 2, 309. CrossRef
11. J. H. Clark, A. P. Kybett, D. J. Macquarrie, S. J. Barlow, and P. Landon, J. Chem. Soc., Chem. Commun., 1989, 1353. CrossRef
12. (a) Y. Izumi, K. Urabe, and M. Onaka, Catal. Today, 1997, 35, 183; CrossRef (b) J.–i. Tateiwa, E. Hayama, T. Nishimura, and S. Uemura, J. Chem. Soc., Perkin Trans. 1, 1997, 1923.
13. M. Jeganathan, A. Dhakshinamoorthy, and K. Pitchumani, ACS Sustainable Chem. Eng., 2014, 2, 781. CrossRef
14. M. Jeganathan and K. Pitchumani, RSC Adv., 2014, 4, 38491.
15. N. N. Binitha, P. P. Silija, V. Suraj, Z. Yaakob, and S. Sugunan, in IOP Conference Series: Mater. Sci. Eng., 2011, 17, 1.
16. M. Frenkel, Clays Clay Miner., 1974, 22, 435. CrossRef
17. O. De Poalis, L. Teixeira, and B. Török, Tetrahedron Lett., 2009, 50, 2939. CrossRef
18. H. O. Jones and P. E. Evans, J. Chem. Soc., Trans., 1911, 99, 334. CrossRef
19. Y.–C. Wu, L. Liu, H.–J. Li, D. Wang, and Y.–J. Chen, J. Org. Chem., 2006, 71, 6592. CrossRef
20. G. M. Badger, B. C. Ennis, and W. E. Matthews, Aust. J. Chem., 1963, 16, 828. CrossRef
21. R. S. Varma, R. Dahiya, and S. Kumar, Tetrahedron Lett., 1997, 38, 2039. CrossRef
22. C. C. Tung, Tetrahedron, 1963, 19, 1685. CrossRef
23. R. ter Halle, B. Colasson, E. Schulz, M. Spagnol, and M. Lemaire, Tetrahedron Lett., 2000, 41, 643. CrossRef
24. A.–H. Li, E. Ahmed, X. Chen, M. Cox, A. P. Crew, H.–Q. Dong, M. Jin, L. Ma, B. Panicker, K. W. Siu, A. G. Steinig, K. M. Stolz, P. A. R. Tavares, B. Volk, Q. Weng, D. Werner, and M. J. Mulvihill, Org. Biomol. Chem., 2007, 5, 61. CrossRef
25. C. M. M. G. Gómez, V. V. Kouznetsov, M. A. Sortino, S. L. Álvarez, and S. A. Zacchino, Bioorg. Med. Chem., 2008, 16, 7908. CrossRef
26. N. Sudhapriya, A. Nandakumar, and P. T. Perumal, RSC Adv., 2014, 4, 58476.
27. Z. Huo, I. D. Gridnev, and Y. Yamamoto, J. Org. Chem., 2010, 75, 1266. CrossRef
28. L. S. Povarov, V. I. Grigos, and R. A. Karakhanov, Russ. Chem. Bull., 1965, 14, 344. CrossRef
29. T. Demaude, L. Knerr, and P. Pasau, J. Comb. Chem., 2004, 6, 768. CrossRef
30. M. Movassaghi and M. D. Hill, J. Am. Chem. Soc., 2006, 128, 4592. CrossRef
31. B. Li, C. Guo, X. Fan, J. Zhang, and X. Zhang, Tetrahedron Lett., 2014, 55, 5944.. CrossRef
32. O. Iwamoto, K. Suzuki, Y. Terao, and M. Sekiya, Chem. Pharm. Bull., 1976, 24, 2409. CrossRef
33. A. I. R. N. A. Barros, A. F. R. Dias, and A. M. S. Silva, Monatsh. Chem., 2007, 138, 585. CrossRef
34. K. K. H. Chandrashekarappa, K. M. Mahadevan, and K. B. Manjappa, Tetrahedron Lett., 2013, 54, 1368 CrossRef