Translational NeuroImaging of the CNS: Novel Pathways to Drug Development
Abstract
Scientists engaged in drug discovery and development face many critical issues along the road to identifying the best drug candidates to bring forward for testing in patients. In neuroscience, these challenges can involve particularly demanding questions regarding target engagement, the predict ability of endpoints in animal models, new disease model validation, CNS penetration, and the identification of pharmacodynamic markers. For neurological conditions such as Alzheimer’s disease, clinical trials of novel drugs that may modify the course of disease, rather than targeting specific symptoms, add extra layers of complexity. Major studies designed to track the course of a disease increasingly depend on noninvasive, translational imaging. In this brief review, we highlight examples of the new wave of neuroimaging studies that engender useful biomarkers of disease for translational research.
INTRODUCTION
Imaging has long been an essential component of drug research. In addition to light and electron microscopy, noninvasive techniques such as magnetic resonance have been used to evaluate safety and sometimes efficacy (1–3). However, it is only recently that the vast potential for translational imaging techniques has been tapped (4–8). The term “translational imaging” represents an emerging field that builds upon validated clinical methodology to provide noninvasive, quantitative information for drug discovery and development. In essence, it posits that non-invasive imaging techniques can be used similarly in animals and humans. Recent advances in high-resolution and high-sensitivity equipment, along with the development of selective biological probes and biomarkers, are rapidly providing new opportunities to investigate drug efficacy and safety (4, 5, 8–10). Depending on the modality used, imaging has applications throughout the entire drug lifecycle, and can provide data for target validation, defining mechanism of action, demonstrating efficacy/safety in both pre-clinical and clinical settings, and applying biomarkers of biological activity. Information gained in these regards can have direct bearing upon the investment of time and resources for phase 2 trials (Figure 1). Imaging studies allow longitudinal measures in the same subject, and recent developments in small-animal scanners can provide translational data for transition from preclinical into early clinical studies. Molecular and functional imaging applications allow direct visualization, localization, and quantification of molecular targets.
Neuroimaging applications across drug discovery and development. Imaging modalities can be selected at multiple points in the drug discovery and development lifecycle.
Many academic and private research institutions have recently established multimodal in vivo imaging facilities for the development of drugs for conditions such as Alzheimer’s disease. Key tools include translational high-field magnetic resonance imaging (MRI), positron emission tomography (PET), and single photon emission computed tomography (SPECT). X-ray based computed tomography (CT) is usually also used in conjunction with PET and SPECT. High throughput fluorescence and bioluminescence optical imaging can also be used in vivo, but translational opportunities are limited at present, and this technique is thus typically reserved to characterize drugs at early stages of development (11–14).
Each imaging technique has its own strengths and weaknesses, and the appropriate use of an imaging modality depends on the specific challenge being addressed. Consequently, different modalities should be seen as complementary rather than competitive (Figure 2). Indeed, multimodal imaging—the synergistic combination of modalities—is rapidly becoming standard practice, as achieved with PET/CT in the clinic; PET/MRI is under development.
The spectrum of neuroimaging techniques: Specialization and complementarity. All imaging technologies utilize specific electromagnetic wavelengths to generate and measure signals. MRI utilizes radio frequencies at the far right of the electromagnetic spectrum and maintains a high static magnetic field to produce images of high spatial resolution. MRI provides anatomical and some physiological imaging. In contrast, fluorescence/bioluminescence and near-infrared optical imaging utilize visible or near-visible wavelengths to produce images of high sensitivity relatively quickly, but the spatial resolution is lower than that provided by MRI. In vivo optical imaging can provide additional information at the molecular level. More energetic and shorter wavelengths are employed in X-ray and CT scanning, which provide excellent anatomical images of high sensitivity but little information at the molecular level. PET and SPECT imaging, which utilize very short wavelengths—in the gamma range of the spectrum—provide excellent sensitivity and yield information about metabolism, molecular targets, and physiology, although spatial resolution may be better provided by other modalities. (See text for discussion of methods and modalities for translation into human studies and therapeutics.)
Magnetic Resonance Imaging
MRI is a versatile imaging method that utilizes a strong, static magnetic field as well as field gradients and a radiofrequency electromagnetic field to produce images based on the magnetization of aqueous protons distributed within an object. Signal intensity is determined by local proton density and MR relaxation time constants, which are particular to the microenvironment and physiological state. MRI can provide versatile tissue contrasts, and can in some instances differentiate diseased tissues from normal tissues and monitor dynamic changes of physiology. The chief advantages of MRI in vivo include excellent spatial (100 μm or less) and temporal (1 sec or less) resolution, particularly for soft tissues (e.g., brain and soft tumors). As a result, MRI has become a standard procedure in the clinic for diagnosis and prognosis of disease (15–18). Moroever, it is increasingly used to translate preclinical findings from small-animal models of disease (19) into human studies, using the same imaging methods.
Our imaging laboratories at Abbott currently operate two preclinical MRI scanners of different magnetic field strengths (4.7 Tesla and 7.0 Tesla) and bore sizes (40 cm and 30 cm), which are suitable for imaging large and small animals across a range of anatomical and functional techniques, as well as in vivo magnetic resonance spectroscopy (MRS) (2, 9, 19–25).
MRI in Confirmation and Diagnosis of Alzheimer’s Disease
In Alzheimer’s disease (AD), structural imaging by MRI has become part of the routine workup of clinical confirmational or differential diagnosis (16). MRI consistently reveals brain atrophy in the entorhinal cortex and hippocampus in human subjects diagnosed with Alzheimer’s disease or mild cognitive impairment (15–18). A strong correlation has been reported between MRI assessment of the severity of medial temporal atrophy and the severity of medial temporal degenerative pathology observed at autopsy (15). In addition, longitudinal studies of patients who initially manifest mild cognitive impairment illustrate that atrophy in the entorhinal cortex and hippocampus, as revealed by MRI, prefaces cognitive decline and conversion to AD (16, 17). The prognostic value of MRI was further established in a three-year longitudinal study of familial AD (26). Fox et al monitored seven individuals with a family history of AD and showed that the three subjects who became symptomatic during the follow-up period were also characterized by faster rates of hippocampal volume reduction (5–10% reduction per year) than those who remained asymptomatic (about 4% reduction per year). In another three-year longitudinal study, forty-three patients with mild cognitive impairment and twenty-eight patients with AD were followed along with fifty-eight control subjects (27). In this case, the annualized rate of hippocampal atrophy was, at 1.8–3.5% reduction of hippocampal volume, most severe among AD patients; the reduction rate for control subjects was 1.1–1.9%, whereas the group with mild cognitive impairment manifested an intermediate reduction rate of 1.6–3.0%. Recently, the Alzheimer’s Disease Neuroimaging Initiative confirmed the predictive value of measuring atrophy of the entorhinal cortex and hippocampus for AD prognosis over a one-year period (84 subjects with mild AD; 175 with mild cognitive impairment; and 139 healthy control subjects) (28). Most indicative of future cognitive decline, even among patients with mild AD, was atrophy in parts of the medial and lateral temporal lobes and in the frontal lobes. In other studies, anatomical imaging by MRI has proven useful for differentiating among distinct pathologies of cognition (29, 30). Volumetric assessment of brain regions through MRI has thus provided biomarkers for diagnosis and disease progression in AD and promises to increase the efficiency of clinical trials (16, 28). It should be noted, however, that the positive correlation between brain volume loss and cognitive impairment may not be observed in some experimental settings [see (15)].
MRI and Animal Models of AD
Transgenic mouse models of AD have become essential for optimizing the imaging approaches to AD pathology in vivo as well as for understanding genotype–phenotype interactions in the disease (31). Although multiple AD mouse models are available, some are useful for emphasizing a single hallmark of AD pathology, whereas others, such as double- or triple-transgenic models, develop more clinically relevant pathology (31). For example, Tg2576 (hAPP695.SWE) and APP London (V717I) mice develop Aβ neuritic plaques (31–33). The P201S tau transgenic mouse model develops Tau pathology (31), whereas the APP/Tau mouse develops amyloid deposits, neurofribrillary tangles, and neuronal loss, and the 3xTg-AD mouse model (APP/Tau/PS1) develops both neuritic Aβ plaques and tangles (31).
The challenge of accurately tracking the development of regional brain atrophy by MRI in mice must not be underestimated, given that the human brain (about 1,500 ml) is three orders of magnitude larger than the mouse brain (about 0.5 ml). Furthermore, the rodent brain offers relatively poor MRI contrast between white and gray matter, so that tissue boundaries may be difficult to define (34). For example, the border between the dorsal hippocampus and neocortex was not visible in a study tracking brain volume in normal and ApoE-deficient mice before and after cerebral ischemia; measurement of volume changes in other areas, such as the caudate-putamen, globus pallidus, or cerebral cortex, were similarly precluded (35). Interestingly, however, mouse dorsal hippocampus can be visualized at high field strength, and its anatomical borders can even be delineated by a manganese-enhanced MRI technique (MEMRI) (36). Despite promising technical advancements in animal imaging, however, the translation of MRI-generated atrophy measurements into the clinic remains challenging. There is no clear evidence of brain atrophy in the time frame during which amyloid plaques are known to develop (37). Clinically relevant models for such studies are urgently needed.
Diffusion Tensor MRI and AD
Whereas MRI affords assessment of brain volume, diffusion tensor MRI (DTI) allows directional measurement, via indirect detection of water diffusion in CNS tissues, of local tissue microarchitecture (38, 39). Recently, DTI has been widely applied to examine the integrity of white matter tracts and to characterize demyelination or abnormalities of white matter associated with AD (40, 41) and schizophrenia (42, 43). For example, it was reported that water diffusion in temporal white matter fiber tracts was significantly lower in AD patients, suggesting a decrease in fiber density (44). Additionally, DTI can distinguish patients with AD from patients with dementia with Lewy bodies (DLB) (45).
Diffusion Tensor MRI in the Development of Animal Models of AD
Animal models of diseases have also been investigated with DTI (46, 47), whereby biomarkers of AD are proposed to serve as efficacy markers for evaluating novel treatments. For example, in studies of transgenic mice that overexpress the amyloid precursor protein (APP) and serve as an AD model, the time-dependent development of AD-like pathology was clearly observable by DTI (47). In addition, DTI data of formalin-fixed brain specimens collected from a mouse model of AD (i.e., the APPsw model) revealed significant changes in the corpus callosum and ventral hippocampal commissure that reflected the time-dependent development of AD-like pathology (46). We have also examined a transgenic mouse model of AD using DTI to examine the connectivity of white matter fiber tracts in the corpus callosum (see Figure 3). The fiber tractography data derived from DTI experiments clearly show that the coherence of oriented fiber tracts in the corpus callosum is diminished in the transgenic mouse, which is consistent with findings reported for AD (43).
Amyloid-beta–specific reduction in cerebral blood volume CBV determined by fMRI. Functional MRI tracks the dose-dependent decrease in cerebral blood volume caused by the Alzheimer’s plaque–associated amyloid peptide Aβ(1–40). The synthetic peptide that contains the reverse amino acid sequence, denoted as Aβ(40–1), does not elicit the dose-related and region-specific decrease in cerebral blood volume that is associated with Aβ(1–40).
Functional MRI
Functional MRI (fMRI) is a valuable tool to study alterations of brain activity in patients as they respond to specific sensory stimuli or perform particular tasks (48, 49). Functional MRI is often considered synonymous with blood oxygen level–dependent (BOLD) imaging; however, the latter is merely one particular measurement in the arsenal of fMRI techniques. BOLD imaging exploits local deoxyhemoglobin as an endogenous contrast agent, relying upon the paramagnetic property of the ferrous iron in the heme group. Thus, changes in cerebral blood flow and blood volume associated with patient activity provides a metric for patient “function.” As novel fMRI measurements abound, there has been considerable and critical examination of the correlated physiological underpinnings of the fMRI signal, especially regarding BOLD. Although BOLD imaging is still in its adolescence, a unique feature of fMRI is that it provides good spatial and temporal resolutions to map brain function in vivo and to follow physiological and pharmacological reactions to clinical applications.
As a translational tool, fMRI is still evolving, particularly with recent advances, such as the imaging of animals under waking conditions (50) and the feasibility of application in drug discovery (2, 9). For example, we have demonstrated the utility of fMRI in awake rodents to measure changes in regional brain activity induced by the administration of drugs, now increasingly known as “pharmacological MRI” (phMRI) (9, 21, 51, 53). Consequently, this approach holds the promise of affording congruency between animal and human studies of drug effects by providing readouts of drug-induced pharmacodynamic activity of novel CNS-targeted compounds.
Functional MRI in Confirmation and Diagnosis of AD
It is believed that the pathophysiological process of AD occurs prior to the onset of clinical diagnosis. Therefore, early therapeutic interventions, based on the identification of symptoms at the onset of AD, might slow and perhaps ultimately prevent the progression to clinical dementia. Recently, fMRI studies of subjects at genetic risk for developing AD or presenting specified cognitive dysfunction have shown altered neural activation in frontal, temporal, and parietal structures; these data suggest that fMRI may provide predictive markers of memory decline (54–57). Further, fMRI has been used to study task-related episodic memory, a cognitive function that is characteristically impaired in early AD. Sperling et al have used fMRI to demonstrate that, compared to cognitively normal age-matched controls, AD patients manifest decreased activation of the hippocampus and related structures within the medial temporal lobe during the encoding of new memories (58). Paradoxically, several studies have also shown that in the prodromal phase of AD, brain activation can increase, although further longitudinal studies are clearly needed (54, 58). Globy et al have investigated the functional competency of specific brain regions and their relationship to specific memory deficits in AD (56). The authors reported that along the cortical ventral visual stream in AD patients, there was graded reduction of activation when patients were presented with novel, as opposed to repeated, scenes; most impairment occurred in the medial temporal lobe and fusiform regions. Such studies indicate the great promise of fMRI for the diagnosis of AD and evaluation of novel treatments.
Functional MRI and Animal Models of AD
APP23 transgenic mice bear a mutated human APP–encoding gene and specifically overproduce gene product within neurons and reproduce the neuropathological alterations associated with Alzheimer’s disease. Brain activity in these animals, in a forepaw electrical stimulation paradigm, has been investigated by fMRI based on cerebral blood volume (59). As the transgenic animals aged, hemodynamic responses appeared to decrease, an effect that was replicated by subjecting the mice to increasing stimulus amplitude. The age-dependent dysfunction in APP23 mice was attributed in part to compromised cerebrovascular reactivity (59). We have shown that Aβ (1-40) significantly decreases cerebral blood volume in a quantifiable, dose-related, and region-specific manner, whereas the reverse peptide, Aβ (40-1), fails to elicit a vascular response (19) (see Figure 4). This technique can be readily applied to preclinical screening in a longitudinal manner for novel drugs or antibodies targeting disease modification.
Hypermetabolism and hyperperfusion in young transgenic mice that model Alzheimer’s disease. PET reveals changes in glucose metabolism (standard uptake volume, SUV) as indicated by the glucose analog [18F]-fluorodeoxyglucose ([18F]-FDG). Transgenic (Tg) and wild-type (WT) mice were imaged over two different age ranges: 7–8 and 19–20 months. Dynamic [18F]-FDG-PET scans were performed on a dedicated Siemens small animal PET/CT system, using Inveon Research Workplace software to quantify [18F]-FDG uptake in the brain. Automated regions-of-interest analyses for PET data utilized CT data to define and include the entire volume inside the skull. Functional MRI experiments were performed on a Bruker 7T scanner. Acetazolamide was used as a stimulus to assess hemodynamic responsiveness.
Positron Emission Tomography
Target engagement can be determined in vivo only by positron emission tomography (PET) imaging [and single photon emission computed tomography (SPECT); see next section]. PET is a noninvasive imaging technique that makes use of a radiotracer that decays by positron emission; the emitted positron eventually combines, in an annihilation reaction, with an electron in the sample, thereby emitting two high-energy photons (or γ rays) at approximately 180 degrees apart, which are detected by coincidence counting in the PET scanner (60). For many tracers, the distance between the point of emission of the positron and the annihilation event is on the order of 0.1–1 mm, thus intrinsically limiting the spatial resolution. This range varies from tracer to tracer: Some radionuclides (e.g., 82Rb or 124I) emit more energetic positrons, which travel further before annihilation, reducing spatial resolution. Thus, lower energy tracers are usually preferred, but much also depends on the half-life of the desired tracer, which can range from seconds (82Rb, 15O) or minutes (11C) to hours (18F, 64Cu) or even several days (124I). Choice of tracer also depends on the application (e.g., 11C is frequently used for labeling small molecules without affecting pharmacological activity). Chief advantages of in vivo PET include high sensitivity (nM concentrations of tracer probe) and the ability to translate preclinical findings in small animal models of disease to human studies, using the same tracer probes and the same imaging technology.
Clinical PET was born in the field of neurology, and clinical PET scanners have been used for a number of years to conduct studies on pharmacodynamics of investigational drugs, brain development, pathology, and neuroplasticity in humans and, to a more limited extent, in non-human primates. Strong possibilities for biomarker development are indicated in the newly available high-resolution micro PET scanners for small animals. Our imaging laboratories at Abbott currently operate a high-resolution (<1.4 mm) Siemens Inveon PET system, dockable to a CT scanner.
Functional PET and AD
In the brain, glucose metabolism provides about 95% of the energy required for proper function (61); during a functional task, neurons increase glucose consumption (61). Because the brain has a limited ability to store glucose, increased cerebral blood flow is needed to deliver the glucose to meet increased metabolic needs (61). Therefore, in the brain, glucose metabolism is coupled to blood flow (62). Astrocytes are the means of transporting glucose from capillaries to neuronal synapses (63). PET allows assessment of cerebral glucose metabolism using [18F]-fluorodeoxyglucose (18F-FDG) (64,65). Resting state glucose utilization levels are decreased in the temporal and parietal lobes and are a characteristic of AD. Moreover, decreased glucose utilization levels correlate with poorer levels of cognitive performance in outcome measures (MMSE or ADAS-cog score) and with the density of senile plaques and neurofibrillary tangles (10, 66, 67). A decrease in synapses is also seen before neuronal death associated with AD (68), arising from decreased glucose transport to synapses. Therefore, the reduction in brain metabolism reflects brain atrophy in terms of a true loss of neurons and synapses (69).
The characteristic pattern of bilateral temporal and parietal brain glucose hypometabolism allows AD to be distinguished from frontotemporal lobe dementia (10, 70). PET glucose metabolism brain mapping also allows the diagnosis of AD in patients who are difficult to characterize by clinical criteria alone (71). For example, PET can show glucose metabolism deficits in patients at risk for AD. Reductions in glucose metabolism and cognitive function are observed in human subjects with the ɛ4 allele of the gene that encodes apolipoprotein E (APOE) (72). The combination of reduced metabolic rates and genetic risk factors provides a means for pre-syndrome AD detection (69). The sensitivity and specificity of PET screening for AD are high. In one study of twenty-two pathologically verified cases of dementia, the sensitivity and specificity of diagnosis based on clinical criteria were 64% and 87%, respectively, compared with sensitivity and specificity based on PET given as 87% and 62%, respectively (69, 71). In another study, the sensitivity and specificity of PET for the diagnosis of AD were 94% and 73%, respectively (73). Perhaps more importantly, the sensitivity and specificity of PET to diagnose AD in the early stages or in mild cognitive impairment has been reported to be 95% and 71%, respectively (73). Furthermore, increasing volumes of data support the prognostic role of PET in AD, with high sensitivity (93%) and specificity (76%) (73). In addition to resting-state brain metabolism assessment, performance-based memory tasks assessed by PET in patients with suspected AD provide a dynamic evaluation on short-memory storage and retrieving. Therefore, functional PET can also provide an objective way to assess the disease pathophysiology status (69). One hypothesis is that FDG PET may fill the gap between amyloid imaging [such as Pittsburg Compound-B (PIB) imaging] and structural MRI (picking up changes in brain metabolism that occur when β-amyloid deposition is under way but not yet advanced enough to cause structural damage) that are described above on anatomical MRI scans (74). Although studies have shown that the binding of PIB to brain sections is highly correlated with total Ab levels and is negatively correlated with CSF Aβ1–42 levels (75), other preliminary reports do not suggest a clear increase of PIB signals in AD subjects during 1- or 2-year periods of follow-up (76). It is clear that additional information is needed to establish the relationship between plaque burden, cognitive function, and MRI measures. Several are ongoing studies with PIB in clinical trials, with therapeutic agents that will elucidate the utility of PIB as a biomarker of amyloid plaque load.
Functional PET and Animal Models of AD
With early detection of dementias such as AD, the possibility of tracking disease progression (67) and developing new disease-modifying therapies to reduce inherent risk is gaining clinical attention (15, 16, 77). Unfortunately, few studies have been conducted in this challenging area, and preclinical applications are particularly needed. To address this gap, we are using high-resolution PET (with FDG; see above) in conjunction with fMRI in a transgenic mouse model of AD (i.e., Tg2576; see above) to assess brain metabolism and vascular compliance, which are impaired in AD. Unexpectedly, seven- to eight-month-old transgenic mice exhibited marked hyperperfusion and increased glucose metabolism at resting state when compared to age-matched wild-type animals. These traits were less marked in mice at nineteen to twenty months of age. Vascular compliance as assessed by fMRI under acetazolamide challenge was equivalent in transgenic and wild-type mice as they aged. The brain hypermetabolism and hyperfusion can be observed in Figure 5, and these results run counter to those anticipated based on clinical experience in AD. In this way, caution must be exercised in defining “clinically relevant” animal models of AD. Although the underlying mechanism responsible for the imaging results of the transgenic mice is not clear, aberrant excitatory neuronal activity and compensatory remodeling of inhibitory circuitry may be contributory factors (78). Interestingly, hypermetabolism is also evident in other transgenic mice (78–80).
Abnormalities of white matter fiber tracts in the transgenic mouse model of Alzheimer’s disease (Tg 2576) indicated by diffusion tensor MRI (DTI). White matter fiber tractography of wild-type (WT) and transgenic (Tg) mice. Structural orientation of corpus callosum (arrows) in WT can be readily observed (connecting the left and right hemispheres), whereas the fiber tracts were highly disoriented in the diseased TG animal. Of note, white matter structural abnormaility was also observed from the DTI study of AD patients.
Receptor Occupancy in AD as Determined by PET
Development of senile plaques and neurofibrillary tangles in the striatum and hippocampus causes dopamine and serotonin receptor loss that can be detected using PET (69), and PET can thus play an important role in relating the loss of neurotransmitter receptors to the effects of AD on memory and cognition. Likewise, the formation of senile plaques and tangles in the basal forebrain and entorhinal areas causes early loss of cholinergic neurons and nicotinic receptors (81). In the normal state, nicotinic receptors are related to acquisition and retention of verbal and nonverbal information, which is the basis for the “cholinergic hypothesis” for the cognitive symptoms of AD (89). The decrease in the number of nicotinic receptors in AD, as reflected through the use of [11C]-nicotine in PET studies, provides the rationale for cholinesterase therapy in treating memory deficits (81). Indeed, PET has proven valuable in trials of memory-sparing agents examined for the ability to promote receptor binding and selective activation of receptors needed in memory retention (69).
Single Photon Emission Computed Tomography
Single photon emission computed tomography (SPECT) makes use of gamma electromagnetic radiation and comprehensive data computation to produce three-dimensional images of tissues. SPECT is now a standard imaging technique in the clinic for diagnosis of human disease. Recently, technical advances have enabled high-resolution functional SPECT imaging of small laboratory animals. Whereas the gamma radiation exploited in PET is produced via annihilation of a positron with an electron (see above), SPECT imaging uses gamma-emitting isotopes (e.g.,99mTc, 111In, 123I, and 131I) as tracers. In SPECT, up to three gamma cameras are rotated around the subject (usually, a full 360 degrees), and image data are acquired at defined points during the rotation (typically, every three to six degrees). The time taken to obtain each projection (i.e., a two-dimensional appraisal at the given angle) is typically fifteen to twenty seconds, for a total scan time of about fifteen to twenty minutes. Multi-headed gamma cameras can provide accelerated acquisition.
Unlike PET, SPECT can be used to detect multiple isotopes simultaneously, provided that gamma rays of differing energies are emitted. The use of multiple isotopes is not viable for PET, because the annihilation of the emitted positron produces gamma rays of consistent energy. However, given the relatively long half-lives of isotopes used in SPECT, generally producing a single photon during the projection period (see above), it is more difficult to determine the origin of the radiation. To approximate the origin of the gamma radiation, SPECT imaging relies on a series of lead collimators, usually arranged in parallel, to determine the direction of flight, a process known as geometric collimation. Spatial resolution can be maximized by “pinhole collimation,” but fields of view are thereby limited to a relatively narrow range. These collimators thus restrict sensitivity such that SPECT systems typically measure a small fraction of the emitted gamma rays. We currently operate a nanoSPECT/CT system (Bioscan Inc.) that achieves superior sensitivity and resolution through utilization of multiple, swappable, micro-pinhole collimators, thus allowing imaging of animals as small as mice or as large as non-human primates.
Receptor Occupancy SPECT in AD
Ideally, SPECT tracers can be used to image the distribution and density of neuronal receptors in the brain and to provide direct evidence of brain penetration and receptor engagement (82). The development of SPECT tracers, however, is not trivial, as a variety of criteria must be considered: stability of labeling; high affinity and selectivity for the targeted receptor; good blood–brain penetration; and suitable metabolic turnover of tracer compound. The availability of SPECT tracers is more limited than that of PET ligands. The nicotinic receptor ligand [123I]-5-I-A-85380([123I]5-IA) has been used to indicate significant reductions in binding activity in cortical and striatal brain regions in AD patients (83). It has also been exploited to evaluate novel treatments for smoking cessation (84). The dopamine transporter ligand [123I]-β-CIT has been used to delineate striatal dopamine nerve terminal loss in patients with Parkinsonism (85). As part of tracer validation studies, we have investigated the specific binding of neuronal nicotinic receptor subtypes using [123I]-5-IA in nicotinic receptor β2 subunit–deficient (knockout) mice. Our results (see Figure 6) show that binding activity was significantly lower in the knockout mice relative to wild-type animals, giving us confidence to commence more complex occupancy studies in larger species such as rat and non-human primates (not shown). The derived dose-occupancy relationships were informative for understanding target engagement in our clinical trials with neuronal nicotinic compounds.
In vivo imaging of the neuronal nicotinic receptor β2 subtype. Knock-out (KO) and wild-type (WT) mice were imaged using the [123I]5-IA SPECT tracer. Specificity of target engagement of the PET/SPCET tracer can be assessed using genetically modified mice. We examined the binding specificity of [123I]5-IA to neuronal nicotinic receptor subtypes in KO (n= 4) and WT (n=2) mice. Fifty minutes after the infusion of 0.5mCi tracer, each mouse was imaged for forty-five minutes. Representative images clearly show the reduction of tracer uptake in KO mice, and quantitative region-of-interest analysis indicated significant decreases in binding activity at both prefrontal cortex (PFC) and thalamus.
Functional SPECT in AD
Regional cerebral blood flow in both animals and humans has been routinely measured by functional SPECT (82, 86). Radionuclides typically used in this manner are mainly lipophilic agents such as [99mTc]-hexamethylpropylene amine oxine (HMAPO) and [99mTc]-ethylene cysteinate (ECD). These SPECT tracers readily across the blood–brain barrier and then become trapped in neuronal cells, thus allowing a relative measurement of cerebral blood flow. More importantly, functional SPECT allows characterization of perfusion abnormalities in disease states. For example, in AD patients, cerebral perfusion is decreased in temporal–parietal regions early during the disease course, extending to frontal regions as the disease progresses (87, 88). Further, imaging of cerebral blood flow by SPECT may provide prognostic value in predicting which individuals with mild cognitive impairment may convert to AD; in a two-year follow-up SPECT study, converters showed reduced cerebral brain flow specifically in the parietal and temporal lobes as well as posterior cingulate cortex (89). Recently, high-resolution SPECT/CT systems have been developed to image small animals and can be used to assess the biological effectiveness of therapeutic candidates (90). We have applied a split-dose SPECT imaging protocol (91) using [99mTc]-HMAPO to evaluate drug-induced changes in cerebral blood flow changes. For example, we found that acetazolamide (30 mg/kg iv), a known vasodilator used to treat seizures, elicits significant increases in blood flow in the prefrontal cortex and cerebellum (see Figure 7). Notably, this imaging approach permits assessments in a region-specific manner over time, and more importantly, may provide a pharmacodynamic biomarker for translational research. We are currently investigating the feasibility of extending this imaging model to non-human primates and humans.
Drug-induced changes in cerebral blood flow evaluated by split-dose SPECT. In the split-dose SPECT study, rats were infused with a first dose of 99mTc-HMAPO at 1 mCi followed by a second dose at 2 mCi; changes in blood flow were then elicited by saline (n=3) or acetazolamide at 30mg/kg iv (n=3). Based on the images acquired from the pre-drug and post-drug periods, mean R-values (indicator of relative drug-induced change in cerebral blood flow) were calculated from specific regions of interest. Our results indicated that, compared to saline-treated rats, ACZ-treated rats show changes in prefrontal cortex (PFC) and cerebellum that reflect the known vasodilatation effect of ACZ. This imaging technique has been widely used in humans, and thus it can serve as a useful translational tool for drug discovery.
CONCLUSIONS
Advances in neuroimaging are extending our insights into brain structure and function and may afford the identification of biomarkers to advance drug discovery and development for neurological diseases. New insights into the structure and function of disease can also drive the selection of patient populations most appropriate for treatment regimes and for studies into experimental therapeutics. Neuroimaging can also be used to assess the utility of animal models of disease and to back-translate pharmacological responses. We have highlighted some of our efforts using imaging to improve target validation. The variety of imaging technologies that we have discussed are complementary—providing the pharmacologist with powerful opportunities to test hypotheses in experimental animals and in humans. Data from such approaches may afford an unprecedented ability to determine parameters that are by definition essential to pharmacology. Although based on new technologies, the design and utility of neuroimaging experiments emanate from the original principles upon which pharmacology was founded.
Acknowledgments
The authors want to thank the many Abbott members of the extended Imaging Team for their considerable and talented contributions to the work presented in this review.
- Copyright © 2009
References
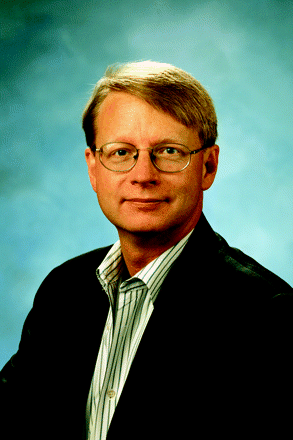
Bryan F. Cox, PhD, is a Director, Advanced Technology, and Head of the Integrative Pharmacology Department at Abbott Laboratories. He received his PhD from the University of Iowa and then pursued a postdoctoral fellowship at the University of Texas Health Science Center. His research has included the therapeutic utility of adenosine receptor agonists for a number of cardiovascular disease states and has over time taken on a focus in integrative pharmacology. At Abbott, he has promoted the growth of imaging from a single MRI scanner into the multimodal imaging facility outlined above. Presently, he leads a multidisciplinary team of pharmacologists studying how novel therapeutics act on major organ systems.
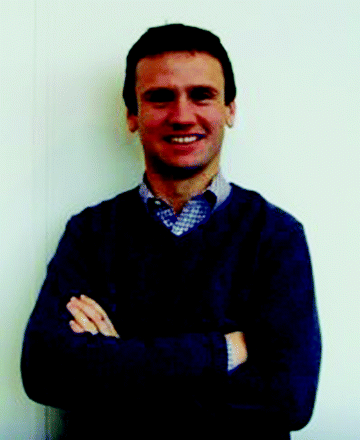
Mark Day, PhD, is an Associate Director at Abbot Labs. He previously led the Psychiatric and Cognitive Disorder Biomarker strategy at Wyeth Translational Medicine in Collegeville, and has pursued research, particularly into Alzheimer’s disease, both in the US and United Kingdom. Currently, he is the Head of Neuroimaging in the Experimental Imaging and Biochemical Biomarkers Department at Abbott Laboratories.
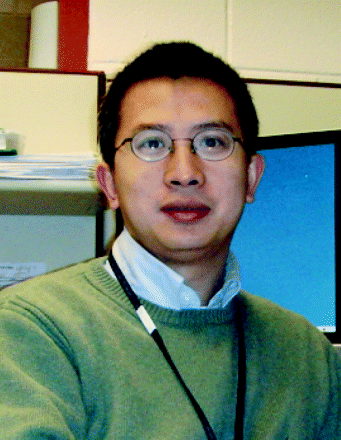
Feng Luo, MD, PhD, received his medical education from West China University of Medical Sciences, after which he served as a neuroradiologist at Beijing Tiantan Hospital. After a fellowship in Medical Physics at the University of Freiburg, Germany, he pursued his PhD at the Medical College of Wisconsin in the Department of Biophysics, functional MRI program, and subsequently has held both academic and industrial positions. He is currently working on the development of new imaging applications for drug discovery and development, with a focus on Alzheimer’s disease.
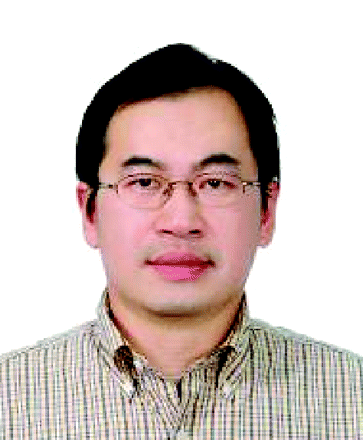
Chih-Liang Chin, PhD, is Senior Scientist in Experimental Imaging at Abbott Laboratories. He received his PhD in bioengineering from the University of Pennsylvania. He joined Abbott in 2004 as a postdoctoral fellow to investigate the application of pharmacological fMRI (phMRI) in awake animals for drug discovery, and since then, he has made significant contributions to establish imaging capabilities for translational use at Abbott with a focus on CNS diseases. His current focus is on the development of translational biomarkers in disease and for the evaluation of potential novel treatments.
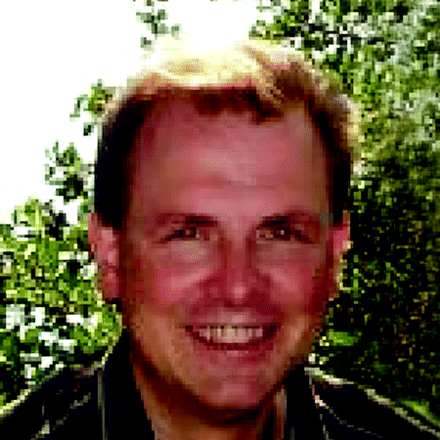
Gerard B. Fox, PhD, is Head of Experimental Imaging and Neuroscience Biomarkers at Abbott Laboratories. He received his PhD in Pharmacology from University College, Dublin, and completed his postdoctoral fellowship at Georgetown University studying mechanisms of neurodegeneration. He has worked extensively across academic, biotech, and pharmaceutical industry environments. He and his team have made significant contributions to the identification, characterization or transition of multiple drug development candidates, with a focus on treatment of neurological disorders. His current focus is on reducing risk for novel drugs across multiple therapeutic areas. Send correspondence to GBF. E-mail gerard.b.fox{at}abbott.com; fax 847-938-5286.