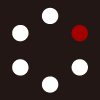
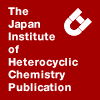
HETEROCYCLES
An International Journal for Reviews and Communications in Heterocyclic ChemistryWeb Edition ISSN: 1881-0942
Published online by The Japan Institute of Heterocyclic Chemistry
e-Journal
Full Text HTML
Received, 29th August, 2009, Accepted, 6th November, 2009, Published online, 9th November, 2009.
DOI: 10.3987/COM-09-S(S)121
■ Synthetic Studies toward Antitumor Sesquiterpenoid Quadrone
Akihiro Ishihata, Megumi Saeki, Masaru Watanabe, Masataka Ihara, and Masahiro Toyota*
Department of Chemistry, Graduate School of Science, University of Osaka Prefecture, Sakai, Osaka 593-8531, Japan
Abstract
A palladium-catalyzed cycloalkenylation and an acid-promoted intramolecular Michael reaction were utilized as the key steps in a synthetic approach to sesquiterpene quadrone 1. This route capitalizes upon the ability of the above reactions to stereoselectively assemble tricyclic core 15 of quadrone 1.Due to the challenging molecular architecture and antitumor activity of sesquiterpene quadrone 1, it has been an interest to synthetic organic chemists, and various approaches to 1 have been reported.1 We first became interested in 1 during our studies on whether our palladium-catalyzed cycloalkenylation2 could effectively construct highly strained tricyclic core 2, which is found in 1. As shown in Scheme 1, we envisaged our retrosynthesis of 1 as follows: palladium-catalyzed cycloalkenylation of silyl enol ether 3 could provide tricyclic compound 2, which could be converted into 1 by functional group manipulations. Compound 3 could be synthesized from acetal 4 via an acid-promoted intramolecular Michael reaction.3 Finally, substrate 4 could be prepared from cyclohexene 5, which could be constructed by an intermolecular Diels–Alder reaction of 1,3-butadiene and (E)-methyl 6-tert-butyldimethylsiloxyhex-2-enoate.
Olefin metathesis between olefin 6 and methyl acrylate in the presence of Grubbs second generation
catalyst afforded (E)-methyl 6-tert-butyldimethylsiloxyhex-2-enoate in 78% yield, which was subsequently subjected to an intermolecular Diels–Alder reaction with 1,3-butadiene to give rise to cyclohexene derivative 5 (88%). Hydrolysis of 5 was accompanied by deprotection of the hydroxyl group to provide the corresponding hydroxyl acid, which was then transformed into unsaturated lactone 74 through iodolactonization followed by elimination. Reduction of 7 with DIBALH (1 equiv.) furnished the corresponding hydroxyl aldehyde, which was treated with acetic anhydride. The resulting aldehyde was reduced with NaBH4 to give alcohol 8. Ozonolysis of 8 and subsequent reductive treatment producedaldehyde 9,
which was converted into requisite unsaturated ketone 10 for the first key reaction via Wittig reaction followed by acetalization (Scheme 2).
Then 10 was treated with a catalytic amount of TfOH. As expected, the intramolecular Michael reaction proceeded stereoselectively to give oxabicyclo[3.3.0]octane compound 11 in 97% yield, which was transformed into hemiacetal 12 by deprotection of the hydroxyl group followed by dehydration5 and hydrolysis. An intramolecular aldol reaction of 12 in the presence of pyrrolidine was accompanied by an intramolecular Michael reaction to afford keto ether 13. To obtain the desired trans-hydrindane derivative for the second key reaction, 13 was subjected to a retro Michael reaction under acidic conditions. After acetylation of the corresponding diol, enone diacetate 144 was isolated in 96% yield. Compound 14 was transformed into the corresponding silyl enol ether, and the subsequent palladium-catalyzed cycloalkenylation successfully synthesized desired cyclization product 154 in 89% yield (Scheme 3).
The relative stereochemistry was established using NOE experiments employing cyclization product 15 as shown in Figure 1.
In conclusion, we demonstrated that 15, a potential intermediate in the synthesis of 1, could be stereoselectively constructed by combining a palladium-catalyzed cycloalkenylation with an acid-catalyzed intramolecular Michael reaction.
ACKNOWLEDGMENT
Part of this research was supported by a Grant-in-Aid for Scientific Research on Priority Areas 18390007 from the Ministry of Education, Culture, Sports, Science and Technology (MEXT).
References
1. Isolation: G. J. Calton, R. L. Ranieri, and M. A. Espenshade, J. Antibiot., 1978, 31, 38.; Recent syntheses of quadrone: (a) A. B. Smith, III, J. P. Konopelski, B. A. Wexler, and P. A. Sprengeler, J. Am. Chem. Soc., 1991, 113, 3533; CrossRef (b) C. G. Sowell, R. L. Wolin, and R. D. Little, Tetrahedron Lett., 1990, 31, 485; CrossRef (c) A. P. Neary and P. J. Parsons, J. C. Soc., Chem. Commun., 1989, 1090; (d) P. Magnus, L. M. Principe, and M. J. Slater, J. Org. Chem., 1987, 52, 1483; CrossRef (e) R. L. Funk and M. M. Abelman, J. Org. Chem., 1986, 51, 3247. CrossRef
2. M. Toyota, T. Wada, K. Fukumoto, and M. Ihara, J. Am. Chem. Soc., 1998, 120, 4916; CrossRef Selected applications to natural product synthesis: (a) M. Toyota, T. Asano, and M. Ihara, Org. Lett., 2005, 7, 3929; CrossRef (b) M. Toyota, M. Sasaki, and M. Ihara, Org. Lett., 2003, 5, 1193; CrossRef (c) M. Toyota, T. Odashima, T. Wada, and M. Ihara, J. Am. Chem. Soc., 2000, 122, 9036; CrossRef (d) M. Toyota, T. Wada, and M. Ihara, J. Org. Chem., 2000, 65, 4565. CrossRef
3. G. Stork and K. S. Atwal, Tetrahedron Lett., 1983, 24, 3819; CrossRef Selected applications to natural product synthesis: (a) M. Toyota, Y. Nishikawa, K. Motoki, N. Yoshida, and K. Fukumoto, Tetrahedron, 1993, 49, 11189; CrossRef (b) M. Toyota, Y. Nishikawa, K. Motoki, N. Yoshida, and K. Fukumoto, Tetrahedron Lett., 1993, 34, 6099. CrossRef
4. Satisfactory analytical data were obtained for all new compounds. Compound 7: IR (neat) 1771 cm–1. 1H NMR (CDCl3, 400 MHz) δ 7.64 (4H, dd, J=8.0 and 1.5 Hz), 7.45-7.35 (6H, m), 6.21-6.15 (1H, m), 5.75-5.70 (1H, m), 4.73 (1H, dd, J=5.3 and 5.3 Hz), 3.68 (2H, td, J=5.9 and 1.5 Hz), 2.74-2.71 (1H, m), 2.51-2.45 (1H, m), 2.31 (1H, ddd, J=11.3, 5.3 and 5.3 Hz), 2.09 (1H, d, J=11.3 Hz), 1.67-1.50 (4H, m) and 1.05 (9H, s). 13C NMR (CDCl3, 100 MHz) δ 179.2, 135.3, 134.5, 133.6, 129.5, 128.5, 127.5, 73.7, 63.4, 42.2, 38.3, 31.2, 30.5, 29.4, 27.0, and 19.3. LRMS m/z 363 (M+–C4H9). Anal. Calcd for C26H32O3Si: C, 74.24; H, 7.67. Found: C, 74.39; H, 7.56. Compound 14: IR (neat) 1738, 1732, and 1682 cm–1. 1H NMR (CDCl3, 400 MHz) δ 7.20 (1Η, δ, J=10.1 Hz), 6.00 (1H, d, J=10.1 Hz), 5.74 (1H, dddd, J=17.1, 9.5, 8.7, and 6.5 Hz), 5.19 (1H, d, J=9.5 Hz), 5.12 (1H, dd, J=17.1 and 1.2 Hz), 5.04-4.97 (1H, m), 4.06 (1H, dd, J=11.4 and 7.4 Hz), 3.99 (1H, dd, J=11.4 and 6.8 Hz), 2.70 (1H, ddd, J=15.1, 9.0, and 9.0 Hz), 2.64-2.48 (4H, m), 2.34 (1H, dd, J=13.4 and 6.5 Hz), 2.09 (1H, dd, J=13.4 and 8.7 Hz), 2.07 (3H, s), 2.06 (3H, s), 1.43 (1H, ddd, J=15.1, 5.7 and 3.2 Hz). 13C NMR (CDCl3, 100 MHz) δ 198.0, 170.7, 170.4, 154.1, 132.3, 130.2, 119.7, 74.8, 66.0, 47.4, 46.5, 40.3, 40.1, 36.5, 33.8, 21.1, and 21.0. LRMS m/z 265 (M+–C3H5). HRMS calcd for C14H17O5 (M+–C3H5) 265.1076. Found: 265.1070. Compound 15: IR (neat) 1738, 1732, and 1682 cm–1. 1H NMR (C6D6, 600 MHz) δ 6.60 (1Η, d, J=9.6 Hz), 5.71 (1H, dd, J=9.6 and 1.5 Hz), 5.31-5.29 (1H, m), 4.84 (1H, s), 4.68 (1H, ddd, J=9.0, 9.0 and 6.6 Hz), 3.86 (1H, s), 3.84 (1H, dd, J=11.4 and 6.0 Hz), 3.70 (1H, dd, J=11.4 and 6.0 Hz), 2.37 (1H, ddd, J=15.0, 9.0, and 9.0 Hz), 2.34 (1H, d, J=9.0 Hz), 1.89 (1H, d, J=17.0 Hz), 1.77-1.72 (1H, m), 1.64 (1H, ddd, J=17.0, 2.4, and 2.4 Hz), 1.59 (3H, s), 1.58 (3H, s), and 1.38 (1H, ddd, J=15.0, 6.6, and 4.2 Hz). 13C NMR (C6D6, 150 MHz) δ 196.2, 170.0, 169.8, 152.7, 143.2, 128.3, 114.2, 75.3, 65.7, 63.6, 59.4, 53.5, 42.0, 40.2, 35.4, 20.4, and 20.3. LRMS m/z 304 (M+). Anal. Calcd for C17H20O5: C, 67.09; H, 6.62. Found: C, 67.05; H, 6.77.
5. (a) K. B. Sharpless and M. W. Young, J. Org. Chem., 1975, 40, 947; CrossRef (b) P. A. Grieco, S. Gilman, and M. Nishizawa, J. Org. Chem., 1976, 41, 1485. CrossRef