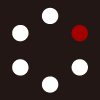
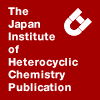
HETEROCYCLES
An International Journal for Reviews and Communications in Heterocyclic ChemistryWeb Edition ISSN: 1881-0942
Published online by The Japan Institute of Heterocyclic Chemistry
e-Journal
Full Text HTML
Received, 29th April, 2013, Accepted, 22nd May, 2013, Published online, 24th May, 2013.
DOI: 10.3987/REV-13-771
■ DIHYDROPYRIMIDINE
Hidetsura Cho*
Graduate School of Pharmaceutical Sciences, Tohoku University, Aramaki, Aoba-ku, sendai 980-8578, Japan
Abstract
The synthesis history, physical properties (stability and tautomerism), ring construction procedures, a variety of reactions, various skeletons (monocyclic, bicyclic, tricyclic, tetracyclic, bicyclo-, and spiro-), and biological activities of dihydropyrimidines are reviewed.CONTENTS
I. INTRODUCTION
II. PHYSICAL PROPERTIES OF MONOCYCLIC DIHYDROPYRIMIDINES
(1) Structure and Stability
(2) Tautomerism
III. SYNTHETIC METHODS OF MONOCYCLIC DIHYDROPYRIMIDINES
(1) Cyclization
(2) Reduction
IV. REACTIONS
(1) O-Alkylation
(2) S-Alkylation
(3) Halogenation
(4) Hydrolysis and Hydrogenolysis
(5) Liebeskind-Srogl, Eschenmoser, and Suzuki-Miyaura Cross-Coupling Reactions
(6) N-Alkoxycarbonylation, N-Acylation, and N-Alkylation
(7) Oxidation
(8) Reduction
(9) Nucleophilic Addition and Nucleophilic Substitution
(10) Ring Cleavage
(11) Rearrangement
V. VARIOUS SKELETONS DERIVED FROM MONOCYCLIC DIHYDROPYRIMIDINES
(1) Bicyclo- and Spiro-Compounds
(2) Bicyclic, Tricyclic and Tetracyclic Dihydropyrimidines
VI. BIOLOGICAL ACTIVITIES
VII. CONCLUSION
I. INTRODUCTION
Biginelli reported the first synthesis of dihydropyrimidines (3,4-dihydropyrimidin-2(1H)-ones) 1a in 1891.1 Since then, many researchers, Kappe,2 Cho,3a Atwal,4 and so on, have studied the reactivity, physical properties, synthetic mechanism and various analogue syntheses of 1 and 2.3b A review on dihydropyrimidines 1 was described in 1993 under the title: 100 years of the Biginelli dihydropyrimidine synthesis by Kappe.2a On the other hand, the synthesis of the other types of dihydropyrimidine 3 was first reported in 1899 by Traube and Schwarz,5 followed by only a few researchers, Ruhemann,6 Dodson,7 Heyes,8 and Silversmith.9 Dihydropyrimidines 1 and 2 could be regarded as keto-forms of the general chemical structure of 3. The reported chemical structures of 3 were initially poorly characterized because of the lack of NMR spectra in those days. Thus, in some cases, the chemical structure is ambiguous and complicated owing to tautomerism and the isomerization of double bonds. Namely, dihydropyrimidines theoretically have nine isomeric mixtures including tautomers, which are oxidized to the corresponding
pyrimidines by atmospheric oxygen.10 Therefore, reports on the synthesis and chemistry of 3 were very limited until those by recent independent research groups of Weis,11 Kashima,12 and Cho3 came out. Since then, the chemistry of such compounds has been clarified and a variety of synthetic procedures for pharmacological active compounds2h,4d,e,3i,13-16 and anti-oxidants for materials science17 have been carried out (See section VI, Biological activities).
Although the review by Weis11d on the Traube and Schwarz dihydropyrimidines 3 appeared in 1986, the synthesis and reaction of 3 have already made progress over the past three decades. Therefore, a new description of dihydropyrimidines 3 may be useful for many future researchers in heterocyclic chemistry and medicinal chemistry. In view of these circumstances, the present review was prepared.
II. PHYSICAL PROPERTIES OF MONOCYCLIC DIHYDROPYRIMIDINES
(1) Structure and Stability
When it has different substituted groups, dihydropyrimidine (DP) could be theoretically represented as nine isomers including tautomers. For convenience in comparing DP isomers, informal names are given in parentheses in Figure 2, except for the IUPAC formal nomenclature. There are two types of dihydropyrimidine in terms of chemical structure, namely, enamines (1,4-DP, 1,6-DP, and 1,2-DP) and imines (2,5-DP and 4,5-DP). A cyclic enamine is usually more stable than its corresponding imine in contrast to acyclic compounds. Therefore, it is supposed that 2,5-DP and 4,5-DP are more unstable than 1,4-DP, 1,6-DP, and 1,2-DP. Thus, imines might isomerize to more stable 1,4-DP and 1,6-DP during preparation, storage or under acidic conditions. In fact, an example conversion from an imine to an enamine will be shown later (Scheme 17, eq. 46). As for the substituent effect, an electron-withdrawing group (R3) at the 5-position and an electron-donating group (R1) could stabilize the DP skeleton (1,4-DP and 1,6-DP),11e and electron-donating groups (R2 and R4) at the 4- and 6-positions could also stabilize the skeleton (1,2-DP and 2,5-DP).11b However, Kashima observed a new tautomeric equilibrium between 4,5-DP and 1,6-DP in 2-dimethylamino-4,6,6-trimethyldihydropyrimidine, and suggested the existence of an imine 4,5-DP isomer as determined by an IR spectrum.12a On the other hand, an imine-enamine tautomerism by which the enamine 1,2-DP isomerizes to the imine 2,5-DP as reported.11c,d,g Weis synthesized various DPs, as will be described later, and studied the stability of DPs. In particular, the isolation of less substituted dihydropyrimidines, for instance, monosubstituted DPs (R1=C6H5, Me; R2=R3=R4=H in Figure 2) was undertaken. Among them, 2-phenyldihydropyrimidine was stable, but 2-methyldihydropyrimidine was decomposed. The dihydropyrimidine (R1=R2=R3=R4=H) without any substituted group was extremely unstable and could not be isolated in pure state.11h
(2) Tautomerism
The [1,3]-sigmatropic tautomerism in the case of amidinic systems may be expressed in Figure 3 (eq. 1). Three classes of tautomeric equilibria can be distinguished according to the molecular structure of amidinic compounds: (1) acyclic (eq. 2), (2) semicyclic (eq. 3), and (3) cyclic or annular compounds (eq. 4). As for the tautomerism of cyclic amidines, studies of a five-membered ring, for instance, imidazoles, were extensively performed.18 As for a six-membered ring, the tautomerism of dihydropyrimidines had not been studied until the report by Weis and Mamaev in 1975.11a A dihydropyrimidine is usually observed as a single compound in an NMR spectrum, but it is actually a mixture of 1,4-DP and 1,6-DP. Proton transfer from one nitrogen atom to the other is very fast and the NMR spectrum usually resembles that of a single compound just like that of an imidazole derivative. Regarding the dihydroform of DPs in solution, Weis suggested that, under certain experimental conditions, solutions of substituted dihydropyrimidines exhibited two independent NMR peaks for the two tautomeric species.11a Subsequently, van der Plas19a,b and Girke20 independently studied the behavior of a variety of dihydropyrimidines. They attempted to obtain the NMR spectra in deuteriochloroform (CDCl3) of each of the tautomers (type a and type b), but failed even at -88 °C.
Weis again studied the tautomerism of 6(4)-methyl-2,4(6)-diphenyl-1,4(1,6)-dihydropyrimidines 4 (R1=R2=C6H5; R3=H; R4=Me) and observed two individual tautomers 4a and 4b (Figure 4) at -50 °C in a dilute CDC13 solution (0.001-0.003 M).11b Because CDCl3 contains a small amount of HCl, which causes the rapid proton transfer between two nitrogen atoms, it should be purified through dry Al2O3 or distilled over P2O5 and then through dry Al2O3.11c Dihydropyrimidine exhibits a characteristic nature in crystalline state. Initially, Weis reported that a tautomeric mixture of 4 existed in only 1,4-DP form in a crystal by X-ray crystallographic analysis, but isomerized to an equilibrium mixture of 1,4- and 1,6-DPs in solution.11b This phenomenon depends on DP derivatives3g,j and is not always observed in all dihydropyrimidines. Subsequently, Cho and Taira carried out an X-ray crystallographic analysis of 4-(2-chlorophenyl)-5-ethoxycarbonyl-2,6-dimethyl-1,4(1,6)-dihydropyrimidine 5 and found it to exist in only the electron-localized 1,4-dihydro form 5a with a flat-boat conformation in crystalline state; however, in solution, 5a isomerized to a mixture of dihydropyrimidines, 5a (1,4-DP; major) and 5b (1,6-DP; minor).3e Also, they obtained a very rare result of the X-ray crystallographic analysis of 6-chloro-4-(2-chlorophenyl)-5-ethoxycarbonyl-2-methyldihydropyrimidine 1/2 HCl salt 6 prepared after extractive work-up under basic condition (Figure 5). The data showed that the unit cell contained two different molecules with Cl atoms: the molecules of the electron-localized 1,4-dihydro form and electron-delocalized form. Both forms were linked by hydrogen bonding around the center of symmetry in the unit cell.3e
As for other examples showing different chemical structures in solid state and solution, the keto-enol tautomerism of curcumin, a natural product, was reported.21 Curcumin is in keto form in solid state, but in an enol form as a major component in solution.
Cho and Iwashita synthesized 7 (R1=CF3; R2=o-NO2C6H4; R3=CO2Pr-i; R4=Me, Figure 4) and observed the individual tautomers 7a and 7b at room temperature in condensed solution (C6D6, 0.123 M),3g although it is generally presumed that tautomers could sometimes be observed at very low temperatures below 0 °C and in highly dilute solutions.19c Moreover, they demonstrated that the nature of the substituted groups (CF3, SMe, C6H5, NMe2, H, Me, i-Pr) at the 2-position affected the observation, whether the signals of individual isomers or the average signals of both isomers were found on an NMR spectrum. An electron-withdrawing group (CF3) at the 2-position afforded independent tautomers (with 1,4-DP as the major tautomer and 1,6-DP as the minor tautomer), because the proton transfer may be slow owing to the low electron density on nitrogen. On the other hand, an electron-donating group (NMe2) afforded only one tautomer, 1,6-DP. This may be due to the stabilizing effect by both the NMe2 group and the electron-withdrawing group, CO2Pr-i.3g Subsequently, Nishimura and Yasui synthesized 8 (R1=SMe; R2=H; R3=CO2Et; R4=Me, Figure 4) and found the individual tautomers 8a and 8b3j (Figure 6) and observed that the ratio of 1,4-/1,6-DP in DMSO-d6 gradually changed by the concentration and temperature (Table 1).
III. SYNTHETIC METHODS OF MONOCYCLIC DIHYDROPYRIMIDINES
1) Cyclization
The construction procedures of dihydropyrimidine skeletons should be classified into five types, as shown in Scheme 1.
(1) Cyclization using urea (Biginelli reaction) (Scheme 1, eq. 6)
Biginelli reported the first syntheses of dihydropyrimidines 1a: 3,4-dihydropyrimidin-2(1H)-ones (R1=C6H5; R2=Et; X=O) (eq. 6) by a one-pot condensation reaction of urea 9a (X=O), an aromatic aldehyde 10, and ethyl acetoacetate 11a in EtOH.1 Many studies have been performed since then and the review2a mentioned above explains the synthesis, reactions and reaction mechanism of dihydropyrimidin-2(1H)-ones.2d,3a Similarly, 3,4-dihydropyrimidin-2(1H)-thiones 1b (R1=H, aryl, alkyl; X=S) were obtained using thiourea 9b instead of urea 9a.3j,4c,22 Alternatively, the thioxo derivatives 1b were obtained by the thiocarbonylation of 1a with P2S5 or Lawesson reagent.
(2) Cyclization using benzamidine, acetamidine and guanidine (Scheme 1, eq. 7 and Scheme 2)
In 1899, Traube and Schwarz firstly synthesized dihydropyrimidines 3. The cyclization of benzamidine 12a (R1=C6H5) with mesityl oxide 13a (R2=R3=R4=Me; R5=H) afforded 4,4,6-trimethy-2- phenyldihydropyrimidine 3a, but the chemical structure of 3a was described to be an imine: 4,5-DP (Scheme 2).5 They also performed the cyclization of guanidine 12b (R1=NH2) with 13a and obtained dihydropyrimidine, suggested to be 2-amino-4,4,6-trimethyl-4,5-dihydropyrimidine 3b. Successively, Ruhemann found that the reaction of 12a with 3-benzylidene-2,4-pentanedione 13b (R2=C6H5; R3=H; R4=COMe; R5=Me) (eq. 7) at 100 °C resulted in the loss of an acetyl group with the formation of methyldiphenyldihydropyrimidine 3c, whose proposed chemical structure was 1,4-DP.6 Dodson and Seyler used the reaction of 12a with various α-benzylidene ketones 13 for the preparation of 6-methyl-2,4-diphenyldihydropyrimidines 3d.7 Thus, the chemical structures were ambiguous in those days because of the lack of NMR instruments.
Weis synthesized various dihydropyrimidines 3 in good yields by this procedure11h and studied their stability and tautomerism. On the other hand, Kashima and Omote synthesized 1,6-dihydro-4,6,6-trimethypyrimidine 3e-k, substituted at the 2-position with phenyl, Me, i-Pr, OEt, SMe, NMe2, and H to investigate the substituent effects on the properties of dihydropyrimidines.12a,b They also transformed SMe-dihydropyrimidine 3i with NaOR in ROH to OMe-dihydropyrimidine 3l.12g Cho and coworkers synthesized various 5-alkoxycarbonyl-4,5-dihydropyrimidin-6(1H)-ones 2 and 5-alkoxycarbonyl-1,4(1,6)-dihydropyrimidines 3 to find pharmacologically active derivatives (eq. 13). The cyclization of 3-substituted 2-alkoxycarbonyl-2-propenoate 14 (Y=H) with 12a-d (R1=C6H5, NH2, NMe2, Me) in the presence of NaOR afforded compounds 2 (R1=C6H5, NH2, NMe2, Me; R2=substituted phenyl, furyl, thienyl, pyridyl, alkyl; R3=Me, Et, i-Pr) (eq. 12), which were generally stable.3b The stereochemistry of the 4 and 5-positions depended on the manner of substitution of a functional group on a phenyl group [trans/cis=3/2-2/1(ortho); 9/1(meta); only trans (para)].3b Similarly, the condensation of alkyl 2-acetyl-3-aryl-2-propenoate 15 (Y=H) with 12 (benzamidine, guanidine, 1,1-dimethylguanidine, or acetamidine) followed by the dehydration of the resulting tetrahydropyrimidine with p-TsOH or Al2O3 yielded a series of 1,4(1,6)-dihydropyrimidines 3 (R1=C6H5, NH2, NMe2, Me, i-Pr, CF3, H, SMe; R2=C6H5, o- or m-NO2C6H4, o- or m-ClC6H4, o-BrC6H4, p-SMeC6H4; R4=Me; R5=OMe, OEt, OPr-i, O-cyclopropylmethyl) in good to excellent yields, except dihydropyrimidines with R1=NH2 and NMe2 (eq. 13).3c,g,i Other dihydropyrimidines with a variety of long chains (R4=CH2OCH2CH2NR6R7) at the 6-position produced by cyclization were also prepared.3c As other examples of cyclization, Atwal and coworkers also synthesized a series of 1,4(1,6)-dihydropyrimidines 3 (R1=SMe, OMe; R5=O-alkyl)4d,24,25 by the condensation of commercially available 2-methyl-2-thiopseudourea sulfate 12h (R1=SMe)4d or O-methylisourea hydrogensulfate 12i (R1=OMe)4a and α-benzylidene-β-keto ester 15 in the presence of NaOAc or NaHCO3, although alkylthiopseudoureas 12j (R1=SCH2C6H4OMe-p) required for this method were obtained by the alkylation of thiourea 9b.4a,d According to Cho’s and Atwal’s reports, many researchers have tried to synthesize a variety of compounds 3. Especially, in the case of
an amino group at the 2-position, an improvement in the yield of 3 (R1=NH2; R2=aryl; R4=alkyl; R5=O-alkyl) was carried out with the aid of a microwave by Kappe.2g Subsequently, various compounds 3 (R1=R2=R4=alkyl; R5=O-alkyl; or R1=SMe; R2=R4=alkyl; R5=O-alkyl)26,27 with alkyl substituents at both the 2 and 4-positions were synthesized. As for dihydropyrimidines with an amide group at the 5-position, amides 3 (R1=substituted NH, aryl; R2=aryl; R4=Me; R5=NH-alkyl,28 NH-aryl29) were provided by direct cyclization (eq. 13).28,29
When the β-substituent (Y) of 14 and 15 is any leaving group instead of a proton (Y=H), the condensation reaction does not give dihydropyrimidines, but it affords their corresponding pyrimidines.
(3) Cyclization using ammonia (Hantzsch type reaction) (Scheme 1, eq. 8)
The four-component condensation reaction of the 1,3-dicarbonyl compound 16 with two moles of ammonia (or ammonium salts) 17 and another carbonyl compound 18 seems to be a convenient approach to preparing 1,2-dihydropyrimidines 19. This reaction is nearly identical to the Hantzsch synthesis of dihydropyridines. The first successful isolation of 1,2-dihydropyrimidines 19 was achieved by Hoffmann and coworkers,23 but their procedure seems to be limited and to depend on the substituted functional groups.
(4) Cyclization of α,β-unsaturated-β-aminoketone with diarylcarbodiimides (Scheme 1, eq. 9)
A new method of constructing a dihydropyrimidine ring was reported by Katoh and Kashima.12d 4,6-Disubstituted-1-aryl-2-arylimino-1,2-dihydropyrimidines 20 (R1, R2=Me, n-C3H7, C6H5, p-ClC6H4) were conveniently synthesized in high yields by the reaction of α,β-unsaturated-β-aminoketones 21 with diarylcarbodiimides 22 in the presence of a large excess of NaH in DMF at -25 °C. Since this synthetic method is limited to the examples given and was not subsequently studied by other researchers, the position of the C-N bond formation of a dihydropyrimidine ring remains unknown (Figure 7).
(5) Cyclization of 1,3-diaza-1,3-butadienes with α,β-unsaturated carbonyl compounds (Scheme 1, eq. 10)
1,3-Diaza-1,3-butadienes are useful for the synthesis of pyrimidinone,30,31,32 heterocyclic fused pyrimidinone,31b-d or pyrimidine,32,33 but have never been used for the synthesis of dihydropyrimidines. The nearly unsolved problem in the field of dihydropyrimidine synthesis concerns the method of synthesizing less substituted dihydropyrimidines.11h-j Cho and coworkers investigated a novel method of constructing a dihydropyrimidine skeleton by the reaction of 1,2,4-trisubstituted-1,3-diaza-4-dimethylamino-1,3-butadienes 23 having an N-protecting group (N-Cbz, N-Boc, N-alkyl, or N-benzyl) with α,β-unsaturated carbonyl compounds 24 such as ethyl acrylate and p-chlorophenyl vinyl ketone.3m,n Consequently, 4-dimethylamino-2-phenyl-1,4,5,6-tetrahydropyrimidines 25 were obtained in good yields. Subsequently, the β-elimination of the dimethylamino group was carried out with MeI in CH2Cl2 or SiO2/molecular sieves 3Å in CH2Cl2 to afford various N-protecting-2,5-disubstituted-1,6-dihydro- pyrimidines 26 in good yields (Scheme 1, eq. 10). Remarkably, the use of phenyl vinyl ketone or 4-chlorophenyl vinyl ketone directly provided 26 in over 90% yield without the formation of tetrahydropyrimidine intermediates 25.3m,n These results were considered to be due to the effective β-elimination of the dimethylamino group of 25 supported by the notion that the high acidity of α-hydrogen at the 5-position was enhanced by the carbonyl group (eq. 10). After the removal of the protecting group, less substituted dihydropyrimidines 26 (X=H) were furnished. The protecting group (R) is attached at the 1-position of 1,3-diaza-1,3-butadiene to stabilize itself as well as to control the reactivity during cyclization.
In summary, the construction procedures for a dihydropyrimidine skeleton should be classified into five types and the positions of the bond formation are drawn in Figure 7. Methods (1) and (2) should be reliable for various derivatives and method (5) may be promising for extensive synthetic research.
2) Reduction
The second synthetic procedure of dihydropyrimidines is reduction using hydrides as reagents; Et3SiH, LiBH4, and LiAlH4. Only a few synthetic examples of dihydropyrimidines (or/and tetrahydropyrimidines) given by the direct reduction of a pyrimidine skeleton were reported. Shadbolt tried the direct reduction of pyrimidines 27 with LiBH4 or LiAlH4, and obtained a mixture of 1,4-dihydropyrimidines 28 and 29 (Scheme 4, eq. 14).34 Weis studied the reduction of pyrimidines 30 having two electron-donating groups (OMe, SMe, OEt, OPr-n, or OBu-n) at the 4- and 6-positions neighboring two nitrogen atoms, and after equilibrium obtained 2,5-dihydropyrimidines 31 (imine-dihydropyrimidines) in 79-95% yields (eq. 15).11e Recently, Shen has also demonstrated the reduction of aminopyrimidine 32 with triethylsilane (Et3SiH) and trifluroacetic acid (TFA) to obtain a 1:1 mixture of 1,6-dihydropyrimidine 33 and 1,4,5,6-tetrahydropyrimidine 34 (eq. 16).35 Kashima and Omote prepared ethyl 1-benzyl-1,4-dihydro-2,6-dimethylpyrimidine-5-carboxylate 35 by the desulfurization of 3612c,e,f under hydrogen for 3 h in the presence of Raney nickel in MeOH at room temperature and compared its nature with that of the corresponding dihydropyridine (Scheme 4, eq. 17).
However, the synthetic procedures for dihydropyrimidines with various substituted groups often give a mixture of dihydropyrimidine and tetrahydropyrimidine. Therefore, to obtain a dihydropyrimidine skeleton, the general cyclization procedures mentioned previously are recommended.
IV. REACTIONS
Conversion from a dihydropyrimidine into another dihydropyrimidine derivative has been extensively studied by a lot of groups, and various reactions have been discovered and developed.
(1) O-Alkylation
The treatment of dihydropyrimidinone 2a-c (R1=Me; R2=C6H5, 2-furyl, 2-thienyl; R3=Et) with Meerwein reagent (Et3OBF4/CH2Cl2) or
2d-e (R1=Me; R2=m-BrC6H4, 2-pyridyl; R3=Me) with dimethyl sulfate and K2CO3 in MeOH afforded imine-dihydropyrimidines 37a-c (R4=Et: 42%, 21%, 23%) or 37d-e (R4=Me: 44%, 39%), respectively (eq. 18).3d On the other hand, the O-alkylation of Biginelli
dihydropyrimidine 1a (X=O) in the presence of NaHCO3 in N,N-dimethylformamide at 70 °C furnished the O-alkylated compound 38 (XR4=O-allyl, OMe; R2=C6H5; R3=Et)(eq. 19).26
(2) S-Alkylation
S-Alkylation at the 2-position of the dihydropyrimidine 1b (X=S) with alkyl halide was performed in the presence of a base (K2CO3 or pyridine) by a lot of research groups to provide the corresponding S-alkylated 1,4(1,6)-dihydropyrimidines 39 (XR4=SMe, SC5H11, SCH2COC6H5, SCH2CO2H etc.; R3=Et) in excellent yields (eq. 19).3g,4d,36-40 As mentioned in the section of cyclization (eq. 13), some of the compounds could be obtained by the direct condensation of S-alkylthiourea with α, β-unsaturated ketoesters.4d
(3) Halogenation
Although the chlorination of carbonyl groups of organic compounds and heterocycles is easily achieved, it is very difficult to obtain chlorinated dihydroheterocycles. Namely, the chlorination of dihydroheterocycles with phosphorus oxychloride (POCl3) usually gives oxidized chloroheterocycles but not chlorodihydroheterocycles.3h For instance, the POCl3 chlorination of dihydropyrazinones 40 (R=C6H5) or dihydropyridazinone 41 afforded chloropyrazines 42 or monochloropyridazine or dichloropyridazine 43 and 44, respectively (eqs. 20, 21).41 Folkers and Johnson42 reported that the chlorination of dihydropyrimidines 45 (R6=H) with POCl3 did not yield the desired dihydropyrimidine derivative, while Khanina reported that the chlorination of N-1 methylated dihydropyrimidines 45 (R6=Me) with POCl3/PCl5 gave oxidized pyrimidinone 46 and chloropyrimidine 47, but not chlorodihydropyrimidine (eq. 22).43 Doehner reported that the chlorination of dihydropyridine 48 with POCl3 afforded the chloropyridine 49 due to the spontaneous air oxidation or
the successive chlorination and dehydrochlorination (eq. 23).44 However, Cho and Ueda reported the first successful POCl3 chlorination at the 6-position of 4,5-dihydropyrimidin-6(1H)-ones 2a-i (R1=Me, NMe2, C6H5; R2=C6H5, substituted C6H5, 2-furyl, 2-thienyl; R3=Et) (eq. 24). Thus, a series of chlorodihydropyrimidines 50a-i were obtained in 27-87% yields.3d Recently, Yasui and Kobayashi have synthesized ethyl 6(4)-chloro-2-methyl-4(6)-phenyl-1,4(6)-dihydropyrimidine-5-carboxylate 50j by the chlorination of the corresponding ketone with POCl3 or phenylphosphonic dichloride (C6H5P(O)Cl2) at 110 °C in 60% yield.3k On the other hand, Zych and coworkers reported a new procedure of introducing a halogen atom into a dihydropyrimidin-2-one skeleton at the 5-position (eq. 25). Namely, they obtained 6-methyl-4-phenyl-5-halo-3,4-dihydropyrimidin-2(1H)-ones 51a-d (Y=Br, I; R2=4-ClC6H5, 4-OMeC6H5, benzyl, cyclohexyl; R4=H) by the halodecarboxylation of carboxylic acids 52a-d (R2=4-ClC6H5, 4-OMeC6H5, benzyl, cyclohexyl; R3=H; R4=H) with oxone (monopersulfate compound: 2KHSO5.KHSO4.K2SO4) and NaI/Na2CO3 in aqueous MeOH.45
(4) Hydrolysis and Hydrogenolysis
The hydrolysis of the esters 3, 50, and 52 was not easily achieved (Scheme 3 and 6).1c,2a,27 For example, Zigeuner showed that N-1 methylated dihydropyrimidin-2-one 52a (R4=Me) was hydrolyzed with an aqueous base to a carboxylic acid derivative, but not the N-1 unsubstituted compound 52b (R4=H) (eq. 25).46 The hydrolysis of benzyl ester 52c (R3=Bzl) was accomplished in excellent yield using AlCl3 in CH2Cl2 at 0 °C to room temperature.45 Also, the hydrogenolysis of benzyl ester 52c with H2/Pd-C in EtOH at room temperature20 and the treatment of 52c with iodotrimethylsilane in CHCl3 at 60 °C were used for debenzylation.
(5) Liebeskind-Srogl, Eschenmoser, and Suzuki-Miyaura Cross-Coupling Reactions
The transformation from the Biginelli-type dihydropyrimidine 1b to the Traube-Schwarz-type dihydropyrimidine 3 was undertaken by Kappe and coworkers. The direct microwave-assisted Pd(PPh3)4-catalyzed/CuTC(copper(I) thiophene-2-carboxylate)-mediated Liebeskind-Srogl C-C cross-coupling reaction2o of 3,4-dihydropyrimidine-2-thiones 1b (X=S; R2=aryl; R3=CO2R; R4=Me) with boronic acids led to various forms of 1,4(1,6)-dihydropyimidine 3 (R1=Ph, 2-phenylvinyl; R3=CO2R; R4=Me) in 14-82% yields.2i,l,o Similarly, they obtained benzoyldihydropyrimidine 3 (R1=C6H5; R2=aryl; R3=COC6H5; R4=Me) from thiolester 1c (X=S; R3=COSEt; R4=Me) (Scheme 7, eq. 26).2k Eschenmoser coupling reactions of 1b with α−bromoacetophenone in the presence of K2CO3/acetone were independently carried out at the same time by the groups of Wyatt26 and Singh37 to provide 2-substituted-vinyldihydropyrimidines 3 (R1=CH=C(OH)Ph; R2=aryl; R3=CO2R; R4=Me) (eq. 26) in 80% yield. The Suzuki-Miyaura cross-coupling reaction on a dihydropyrimidine ring was reported by Zych.45 The cross-coupling reaction at the 5-position of dihydropyrimidines 53 (X=Br, I) with organoboronic acids in the presence of 5 mol% Pd2(dba)3, Pd(t-Bu3P)2, or PdCl2(dppf) and a base (Na2CO3, NaOBu-t, or KF) provided 6-methyl-4-phenyl-5-substituted-3,4-dihydropyrimidine-2(1H)-ones 54 from 5-halodihydropyrimidines 53 (R1=C6H5, 4-ClC6H5, 4-OMeC6H5, benzyl, cyclohexyl; R2=C6H5, m-, p-MeC6H4, m-, p-OMeC6H4, 2-naphtyl, 2-phenylvinyl) in 46-92% yield (eq. 27). However, sterically hindered or electron-deficient organoboronic acids (R1=C6H5; R2=o-MeC6H4, p-FC6H4) gave generally inferior results. At the same time, Yasui and Kobayashi developed the Suzuki-Miyaura cross-coupling reaction at the 4-position of dihydropyrimidine 55 (X=Cl) (see the section of N-alkoxycarbonylation) with organoboronic acids or triethylborane in the presence of Pd catalysis, although the reaction of 3 (R3=CO2Et) with organoboronic acids did not work out.3k The reaction of 55 and organoboronic acids or Et3B in the presence of 5 mol% bis(triphenylphosphine)palladium (II) dichloride: PdCl2(PPh3)2 and Cs2CO3 in DMA at 60 °C provided 56 (R1=C6H5; R2=C6H5, o-MeC6H4, o-OMeC6H4, 2-phenylvinyl, 3-thienyl, Et) in 85-99% yield. However, electron-deficient organoboronic acid afforded 57 (R1=C6H5; R2=p-NO2C6H4) in 69% yield (eq. 28).
The cross-coupling reactions at the 2-, 4- and 5-positions should be useful because they might be applied to synthesize other dihydropyrimidines with various functional groups at all positions.
(6) N-Alkoxycarbonylation, N-Acylation, and N-Alkylation
Novel methods of regiospecific N-alkoxycarbonylation, N-acylation, and N-alkylation of dihydropyrimidines 57 for obtaining pharmacologically active compounds, calcium antagonists, were discovered in 1985 by Cho and coworkers (Scheme 8).3c The reactions of sodium salts of 57 with alkyl chloroformate (ClCO2R4) or acyl chloride (R5=Me, cyclopropyl) regiospecifically yielded a single compound, 58 (R3=Et, cyclopropylmethyl; R4=Me, Et, (CH2)2OMe), in good to excellent yields. Similarly, 58 (R1=Me, n-C7H15) was obtained from the alkylation of its sodium salts of 57 and alkyl halide (MeI, C7H15I)/HMPA in moderate yields (eq. 29). The finding of the regiospecificity could be rationalized in two ways. First, the nitrogen atom at the 3-position may be less sterically hindered since the DP ring can be assumed almost perpendicular to the phenyl ring. Secondly, if one draws the possible resonance structures of the anion that would result from the removal of a proton from the nitrogen of 57, it is clear that N-3 should bear a higher electron density than N-1 and therefore should be more nucleophilic.3c To obtain dihydropyrimidines with a complicated alkoxycarbonyl group or a nitrogen-containing alkoxycarbonyl group at the 1-position, the N-alkoxycarbonylation of 57 with trichloromethyl chloroformate (phosgene dimer) was performed.3f Thus, without isolating, the resulting unstable intermediate 59 was treated with alcohol (R4OH) or aminoalcohol to provide the compounds 60 (R1=o- or m-NO2C6H4, 2-3-Cl2C6H3; R3=i-Pr, cyclopropylmethyl; R4=(CH2)2-2-pyridyl, (CH2)2N(Bzl)(CH2)3Ph, etc.) in good yields (eq. 30).3f
On the other hand, the N-alkoxycarbonylation of Biginelli dihydropyrimidines 61 did not regiospecifically give the desired N-3 substituted compounds 62 as will be described later (Scheme 9, eq. 31).3a Therefore, Atwal and coworkers synthesized a series of N-3-substituted dihydropyrimidines 624c,d via 63 and 64 to synthesize pharmacologically active compounds according to the cyclization procedure3c (eq. 32). Thus, dihydropyrimidines 64a (X=OMe) and 64b (X=S-p-methoxybenzyl) were obtained in good yields, for comparison with the dihydropyridines, calcium antagonists.4c-e The deprotection of 64b (X=S-p-methoxybenzyl) was accomplished by treatment with an aqueous acid to furnish a series of 62 (R1=CO2Et etc; R2=NO2C6H4, ClC6H4, CF3C6H4; R3=Et, i-Pr; R4=H; Z=O, S) (eq. 33).4d They found a potent compound, 62a (R1=CO2Et; R2=m-NO2C6H4; R3=i-Pr; R4=H; Z=S), among them.4d-g Only a few studies on the synthesis of optically active compounds were reported.11k Atwal and Rovnyak obtained the optically active enantiomers 63a and 65b by transformation from 63a, as shown in Scheme 10.4e,f Consequently, the compound 63a was converted to the diastereomeric ureas 66a and 66b by a two-step procedure involving the treatment of 63a with p-nitrophenyl chloroformate followed by the reaction of the resulting intermediate with (R)-(-)-α-methylbenzylamine 67 (eq. 34). The diastereomers 66a and 66b were separated by crystallization. The cleavage of the urea group was performed by treatment with DBU to yield the enantiomers 68a and 68b, which were transformed via 69 to yield 65a and 65b, respectively. The absolute stereochemistry of 65a was confirmed by single-crystal X-ray analysis.
As for the reactions of Biginelli dihydropyrimidine, 61b (R2=aryl; R4=H; Z=O) was alkylated regioselectively at the N-1 position when treated with alkyl halide in the presence of a suitable base (Scheme 9).3a,47 On the other hand, the N-acetylation and N-formylation of 61b with acetic anhydride2c,42b and DMF/POCl32c occurred at the N-3 position to give the compounds 62b (R1=Ac; R4=H; Z=O) and 62c (R1=CHO; R4=H; Z=O), respectively. As for the N-alkoxycarbonylation of 61 (R4=H; Z=O), Cho and coworkers reported that the regioselectivity of the products depended on the position (ortho, meta) of the substituent on the aromatic ring (R2) of 61. Namely, ortho-substituted compounds resulted in N-1 alkoxycarbonylation, because of the steric hindrance of a nitro group (eq. 31).3a To the contrary, the N-alkoxycarbonylation of meta-substituted compounds (R2=m-NO2C6H4; R3=Et; R4=H; Z=O) with NaH/ClCO2Et (or ClCO2C7H15-n) afforded N-3-substituted dihydropyrimidines 62d and 62e (R1=CO2Et, R1=CO2C7H15-n; R2=m-NO2C6H4; R3=Et; R4=H; Z=O) in 80-99% yield as a single compound, while the reaction of 61 (R2=o-NO2C6H4; R3=Et; R4=H; Z=O) yielded the N-1-substituted compounds 62f (R1=H; R2=o-NO2C6H4; R3=Et; R4=CO2C7H15-n; Z=O) in 10% yield with the recovery of 61f. To obtain the N-3-substituted compound 62e with o-NO2 derivatives, protection with a methoxymethyl (MOM) group at the 1-position of 61e (R2=o-NO2C6H4; R3=cyclopropylmethyl; R4=H; Z=O) was effective. Thus, the compound 62e (R1=CO2C7H15-n; R2=o-NO2C6H4; R3=cyclopropylmethyl; R4=H; Z=O) was provided in 40% yield (3 steps) from 61e after protection with the MOM group, alkoxycarbonylation and the removal of the MOM group with an aqueous acid.3a
(7) Oxidation
Dihydropyrimidines are sometimes spontaneously oxidized during SiO2 column chromatograpy or storage. The reported method for the chemical oxidation of 4-substituted-1,4(1,6)-dihydropyrimidine 3 utilizes mainly transition-metal-based oxidizing agents, such as Mn(OAc)3,48 MnO2,2g (NH4)2Ce(NO3)6,2g and CuCl2/Na2CO3/t-BuOOH.49 The dichlorodicyanoquinone (DDQ, benzene, reflux, 1h) oxidation of 6(4)-chloro-1,4(1,6)-dihydropyrimidine 3 (R1=C6H5; R2=p-SMeC6H4; R3=Et; X=Cl) furnished the corresponding pyrimidine in 88% yield, but 3 (R1=NMe2; R2=m-NO2C6H4; R3=Et; X=Cl) gave the pyrimidine in 38% yield.3d Recently, mild and efficient oxidation of 2-methylthio-4-alkyl- or 4-aryl-1,4-dihydropyrimidines 3 with (diacetoxyiode)benzene: PhI(OAc)2 has been reported.40 Also, an efficient aerobic oxidative dehydrogenation of 4-substituted dihydropyrimidinones 1 and dihydropyrimidines 3 was performed to give the corresponding pyrimidinones and pyrimidines, respectively, in high yields by molecular oxygen (1 atm) in the presence of catalytic amounts of N-hydroxyphthalimide (NHPI) and Co(OAc)2.27
(8) Reduction
Various methods of reduction to furnish 1,4(1,6)- and 2,5-dihydropyrimidines from pyrimidines were described above (Scheme 4, eqs. 14-17). However, only a few examples exhibit the reduction from a dihydropyrimidine to a tetrahydropyrimidine. In the case of having an N-alkoxycarbonyl substitute at the 3-position as 70, the reduction of 70 hydrochloride (prepared with anhydrous HCl/Et2O in MeOH)
with 2 mol equivalents of NaBH4 at room temperature for 0.5 h quantitatively furnished the corresponding tetrahydropyrimidine 71a (X=o-NO2, R1=Me, R2=CO2Et) or 71b (X=o-NO2, R1=C6H5, R2=CO2Me) as the sole compound (Scheme 11, eq. 35).3c The stereochemistry of 71a was determined as a cis stereoisomer by X-ray crystallographic analysis. In the case of the acyl compound 70 (R1=Me, R2=COMe), the NaBH4 reduction gave a complex mixture. However, in the case where N-3 was unsubstituted or alkyl-substituted, the reduction afforded a 1:1 or 4:1 mixture (the major isomer was cis by an NOE) of stereoisomeric tetrahydropyrimidines 70c (X=m-NO2, R1=Me, R2=H) or 70d (X=o-NO2, R1=Me, R2=n-C7H15) in each case. On the other hand, Kashima and coworkers performed the reduction of 1,4-dihydro-4,6-dimethyl-1-phenylpyrimidine 72 with a large excess of NaBH4 in EtOH at room temperature for 24 h and obtained 4-anilino-2-methylaminopentane 73 via hexahydropyrimidine 74 (eq. 36).12h
(9) Nucleophilic Addition and Nucleophilic Substitution
Heyes reported that the reductive nucleophilic addition at the 6-position of 4-methyl-2-phenylpyrimidine 75 with phenyllithium provided 4-methyl-2,6-diphenyl-1,6-dihydropyrimdine 76 (eq. 37).8 Akiba and coworkers observed that the reaction of pyrimidine 77 quarternized with acetyl chloride (1 mol equivalent) and trimethylsilyl ether 78 (1 mol equivalent) of enolic acetophenone afforded 1,2,3,4-tetrahydropyrimidine 79 in 48% yield as a mixture of diastereoisomers without the 1:1 adduct 80 (eq. 38).50
The reactivity at the 2-position of dihydropyrimidines is quite different from that of pyrimidines. In fact, compared with well-known nucleophilic substitutions of pyrimidines having a leaving group at the 2-position,2g,51-54 those of dihydropyrimidines are rare because of the low reactivity of dihydropyrimidines at the 2-position. Actually, Kwon’s theoretical study revealed that the LUMO energy level of dihydropyrimidines such as 2-chlorodihydropyrimidine-5-carboxylic acid was higher at about 11.8-17.5 kcal/mol than that of 2-chloropyrimidine-5-carboxylic acid. This high-lying LUMO energy level of dihydropyrimidines induced a barrier for attack by the HOMO of nucleophiles such as aniline.3j
Thus, only a few studies on the substitution at the 2-position of 4-phenyldihydropyrimidines have been reported.2g,4c,d,55,56 Moreover, the yields are not always satisfactory. Namely, Atwal reported the synthesis of 2-amino-4-(3-nitro)phenyldihydropyrimidines 82 (Scheme 12, eq. 39) in 30-54% yield by aminolysis with NH3 or MeNH2 at the 2-position of 1,4(3,4)-dihydropyrimidines 83.4c,d
Kappe obtained 2-benzylamino-1,4-dihydropyrimidine 84 in 58% yield by the microwave irradiation of a mixture of the 2-methylthio-1,4-dihydropyrimidine 85 TFA salts in DCM and benzylamine at 120 °C (eq. 40).2g Overman reported the synthesis of 2-aminodihydropyrimidine 86 (X=H, m-NO2) in 75-77% yield by aminolysis (NH3–NH4Cl, 70 °C, 12 h, in a sealed tube) with 87 having a pyrazole moiety at the 2-position (eq. 41).56b Recently, Nishimura and Yasui have found more effective procedures in which the nucleophilic substitution at the 2-position of 2-methylsulfanyl-1,6-dihydropyrimidine-1,5-dicarboxylate 88 with aromatic or aliphatic primary amines (aniline, 4-methoxyaniline, 4-methoxycarbonylaniline, benzylamine, n-hexylamine, O-benzylhydroxylamine, etc.) provided a variety of 6-unsubstituted-2- amino-1,6-dihydropyrimidines 89 in 76-97% yield (eq. 42).3j The deprotection of a Boc group in a series of compounds 89 was quantitatively performed using TFA. Interestingly, in contrast to 88, the other compound 90 protected at the 1-position with a Boc group gave no substituted dihydropyrimidines (eq. 42) except ring-cleaved compounds, as will be described
later. The methods of synthesizing 88 and 90 from 91 were somewhat tricky because a Boc group was regioselectively introduced at the different positions in 90 and 92. Consequently, the compounds were provided via intermediates 92 and 93, respectively (Scheme 14).
(10) Ring Cleavage
Generally, most heterocyclic rings cannot be easily cleaved and so only a few studies on them have been reported.12i,57,58 For instance, Maddaluno reported that treating N-substituted pyrrolidines and piperidines bearing an allylic chain α to nitrogen with strong bases led to the opening of a heterocycle and provided 1,3-dienes disubstituted with an alkoxy and an aminoalkyl chain.57 Kashima and Omote described the ring-cleavage of 1,4-dihydro-4,6-dimethyl-1-phenylpyrimidine 72 with a large excess of NaBH4 in Scheme 11 (eq. 36).12h A novel method of the [4+2]cycloaddition of 1,6-dihydro-2-dimethylamino- 4,6,6-trimethylpyrimidine 94 as azadienes with acetylenic compounds was also reported by Kashima and Shimizu.12i In this method, the cycloaddition of 94 with dimethyl acetylenedicarboxylate in benzene at room temperature took place across the C-2 and C-5 carbons to furnish the [4+2]adduct 95a, although it was not detected. Retro-cycloaddition also took place with the loss of 2-iminopropane to afford the 2-dimethylaminopyridine derivative 96 with aromatization in 61% yield. Although the dihydropyrimidine 94 consisted of tautomeric mixtures of 94a (1,6-DP) and 94b (4,5-DP), the reaction proceeded via 95a from the tautomer 94a (enamine-DP) but not the other unstable tautomer 94b (imine-DP), because 94b should give the intermediate 95b.
Cho and Yamaguchi observed that the nucleophic addition of 4,6-unsubstituted-1,4-dihydropyrimidine 90 with an aniline derivative or phenylhydrazine in the presence of pyridinium p-toluenesulfonate (PPTS) resulted in a ring cleavage between the 1- and 2-positions of the 1,4-dihydropyrimidine skeleton to furnish the compounds 97a-f (R’=C6H5, p-MeC6H4, p-MeOC6H4, o-MeC6H4, m,p-(MeO)2C6H3, NHC6H5) in 65-95% yield (97g-h; except o-MeC6H4 and NHC6H5 each in 24%),3l although the nucleophilic substitution of 4-methyl-6-unsubstituted-1,6-dihydropyrimidine 88 with the same amines furnished conventional substituted products 89 at the 2-position in excellent yields.3j
The reactions were limited to those of the aniline derivatives above or phenylhydrazine, because aralkylamines, alkylamines, or heterocyclic amines did not cleave the skeleton. The ring-opening chemical structure of 97a (R’=C6H5) was confirmed by X-ray crystallographic analysis.
The reaction proceeded not as in B but as in A (Figure 8) to yield the ring-opening compound 97, presumably by the effect of an α,β-unsaturated ester group and a Boc group. On the other hand, it is assumed that, in the case of reactions of 88 with amines, elimination occurred to afford 89 (eq. 42) so that the product shown in C in Figure 8 generated α,β- and γ,δ-conjugated double bonds.3l
(11) Rearrangement
Only a few reports have been made on the rearrangement of a dihydropyrimidine ring. In particular, van der Plas discovered the di-π-methane photochemical rearrangement from 4,6-diphenyl-1,6-dihydropyrimidine 98 to 5,6-diphenyl-1,2-dihydropyrimidine 99.59 There are two interconversion between 1,4-, 1,6-, and 4,5-dihydropyrimidines and between 1,2- and 2,5-dihydropyrimidines (Figure 2). Although thermal interconversion between two groups was not observed, the photochemical rearrangement of 1,4(1,6)-dihydropyrimidine to 1,2-isomers was observed. The rearrangement should include 2,4-diazabicyclo[3.1.0]hex-2(3)-ene 100, after the successive cleavage of the three-membered ring with a concomitant homo[1,5]hydrogen shift from nitrogen to carbon at the 2-position yielded the imine 101, which isomerized to a rearranged enamine 99 (eq. 46). However, the isolation of 99 failed because of its instability. Therefore, evidence of its existence was only based on spectral and chemical data.
The reaction of 4-chloromethyldihydropyrimidine 10260a with nucleophilic reagents (NaOMe, KCN, NaBH4, and Grignard reagents) caused ring cleavage to afford 2,3,6,7-tetrahydro-2-oxo-1H-1,3-diazepine-5-carboxylates 103 (X=OMe, CN, H, alkyl, aryl) in high yields.60a,b,61-64 Some compounds were converted into 1-carbamoylpyrrole 104 under acidic61 or basic condition.60a On the other hand,
the treatment of 102 with a secondary amine provided 105,60a which was transformed under acidic condition to 1-carbamoylpyrrole 104.
V. VARIOUS SKELETONS DERIVED FROM MONOCYCLIC DIHYDROPYRIMIDINES
(1) Bicyclo- and Spiro-Compounds
In some cases, the reaction (eq. 6) of 9, 10 and 11 or the cyclization (eq. 7) of α,β-unsaturated carbonyl compounds with benzamidine 12a provided bicyclo-compounds. For instance, after the formation of a dihydropyrimidine ring, an ortho-OH group of a benzene ring attacked a double bond to give 2,4-diaza-6,7-benzo-8-oxabicyclo[3.3.1]nonane-2-thione 10620 and 1-methyl-2,4-diaza-6,7-benzo-8- oxabicyclo[3.3.1]non-3-ene 10711l in 79% and 90% yields, respectively (Figure 9). When a large excess of the acylating agent (2,2,2-trichloroethyl chloroformate) was used instead of ethyl chloroformate in the reaction (Scheme 12; eq. 38), 3-phenyl-8,9-bis-(2,2,2-trichloroethoxycarbonyl)-2-oxa-8,9-diazabicyclo- [3.3.1]nona-3,6-diene 108 was furnished in 29% yield from the intermediate 81.50 The bicyclo-compound 109 was obtained by intramolecular Michael addition of a sulfur atom (X=S) of 103 to an α,β-unsaturated carbonyl moiety.60a,b,61
Only a few studies on the synthesis of spiro-dihydropyrimidine derivatives were reported. As shown in Scheme 18, the N-alkylation of 6-bromomethyldihydropyrimidine 11065 with 2-aminopyridine afforded the salt of pyridine nitrogen, and an amino group attacked the β-position of the α,β-unsaturated ester moiety to provide spiro-compound 111.
(2) Bicyclic, Tricyclic and Tetracyclic Dihydropyrimidines
Various methods of synthesizing bicyclic, tricyclic and tetracyclic dihydropyrimidines were developed by many researchers.2-4,12,24-26,36,40,48,56,66-108 The main purpose was to stabilize monocyclic 1,4(1,6)-dihydropyrimidine skeletons and find new biologically active compounds. The synthetic procedures for multicyclic dihydropyrimidines are essentially almost the same as those for monocyclic compounds. In some cases, those compounds were synthesized by the cyclization of the functional groups of neighboring positions or by reactions with any suitable substances at a given position. Therefore, N1-C2,2n,12g,56e,68 C2-N3,2c,3j,3l,4h,i,48,104-108 N1-C2/C5-C6,2m,24,25,75,79,89,95,102 N3-C4,56 N3-C4/C5-C6,88 C5-C6,2h,26,36,40,70-72,76,97-99 C6-N1,77 C4-C5/C5-C6,56 and C2-N3/N3-C456,66 bonds are shared in a fused ring. Thus, bicyclic, tricyclic and tetracyclic dihydropyrimidines were synthesized as shown in Figure 10. Because of the limited space for this review, the chemical structures and compound numbers with reference numbers are simply given in Figure 10.
VI. BIOLOGICAL ACTIVITIES
Investigations on the synthesis of dihydroheterocycles (dihydroazines) such as dihydropyridine,79 dihydropyridazine,80,81 dihydropyrimidine,3,4 dihydropyrazine,82-84 dihydropyran,85 and dihydrothio- pyran73 were intensively carried out to find compounds with a variety of biological activities.3i For instance, 3,4-dihydropyimidin-2(1H)-ones 12a synthesized by Biginelli exhibited antiviral,74,86 antitumor,87 antiinflammatory,67,88 analgesic88 activities, and properties of a platelet-activating factor (PAF) antagonist.78 Khanina and coworkers discovered the cardiovascular activity of N-1-unsubstituted 3,4-dihydropyrimidin-2(1H)-ones 1 in 1978.89 Particularly, a lot of pharmaceutical companies all over the world studied the synthesis of dihydropyridines for the development of clinical candidates having cardiovascular activity. Dihydropyrimidines and dihydropyrazines82-84 were regarded as aza-analogs of the dihydropyridines, such as nifedipine and nicardipine. Therefore, Cho’s3a,c,i and Atwal’s4d-f groups independently reported the synthesis of dihydropyrimidines 58 and 62 showing calcium antagonistic activity. Namely, Cho and coworkers found the regiospecific N-alkoxycarbonylation at the 3-position of Traube-Schwarz 1,4(1,6)-dihydropyimidine 57 (Scheme 8: eq. 29, 30) and successively achieved N-alkoxycarbonylation at the 3-position of Biginelli dihydropyimidine 61 (eq. 31).3a,c,i Although N-substituted 1,6-dihydropyrimidines 60 have an endocyclic π electron system in a dihydropyrimidine ring, N-substituted dihydropyrimidine-2(1H)-ones 62 have an exocyclic π electron system on a dihydro- pyrimidine ring. Therefore, they synthesized N-3-substituted dihydropyrimidine 62e (R1=CO2Et, CO2C7H15-n; R2=m-NO2C6H4; R3=Et; R4=H; Z=O) and N-1-substituted compound 62f (R1=H; R2=o-NO2C6H4; R3=Et; R4=CO2C7H15-n; Z=O). After detailed studies on the structure-activity relationship of a series of compounds 58, 60, and 62, the compound 144 exhibited not only more potent and longer lasting vasodilative action but also a more hypotensive activity with a slow onset than dihydropyridines (nifedipine and nicardipine).3i Moreover, 144 was less effective in blocking atrioventricular conduction in dogs and less toxic than dihydropyridines (nicardipine and nilvadipine). On the other hand, the N-3 substituted dihydropyrimidine 62e showed a more potent and longer lasting vasodilative activity but the N-1-substituted compound 62f did not show any activity.
On the other hand, Atwal and coworkers synthesized a series of N-3 substituted dihydropyrimidines 62.4d-g After extensive studies of
the structure-activity relationship, they finally found one of the most potent compounds, dihydropyrimidin-2-one 145 [(R)-enantiomer],4f which has comparable pharmacological profiles to amlodipine.
Recently, 3,4-dihydropyrimidine-2(1H)-thione 146 (monastrol)90, 91 has been reported as a kinesin spindle protein inhibitor.92 Monastrol arrests mammalian cells in mitosis with monopolar spindles. In vitro, it specifically inhibits the motility of the mitotic kinesin Eg5, a motor protein required for spindle bipolarity.93 Therefore, monastrol is a particularly useful tool for studying mitotic mechanisms.92 A series of bis dihydropyrimidine compounds 147a-f (R=C6H5, 4-FC6H4, 2-ClC6H4, 2,6-diClC6H3, 4-NO2C6H4, furyl) were synthesized and evaluated for their antimycobacterial activity.93 Among them, 147b (R=4-FC6H4) exhibited the most potent activity against M. tuberculosis H37 Rv and INH resistant M. tuberculosis with minimum inhibitory concentrations (MICs) of 0.08 and 0.10 µM, respectively. Emmacin 148, an anti-MRSA agent discovered from a diversity-oriented synthesis, represents a new structural subclass of bacterial dihydrofolate reductase inhibitors.94 Moreover, potent, selective and orally bioavailable dihydropyrimidine inhibitors 149 of Rho kinase (ROCK1) as potential therapeutic agents for cardiovascular diseases have recently been reported.95
In materials science fields, the inhibition and adsorption effects of 5-benzoyl-4-(substituted phenyl)- 6-phenyl-3,4-dihydropyrimidine-2(1H)-thiones (and -ones) on the corrosion behavior of austenitic stainless steel in 0.5M H2SO4 were studied by electrochemical methods. Among them, 150 was found to be a good anticorrosion agent for stainless steel.17
VII. CONCLUSION
In this review, the synthesis history, physical properties (stability and tautomerism), ring construction procedures for dihydropyrimidine skeletons, various reactions from dihydropyrimidines, and biological activities of monocyclic dihydropyrimidines are described in detail. The modification of substituents on the dihyropyrimidine skeleton should be further performed in future research, as well as efforts to stabilize such a skeleton. However, despite the many biological active compounds synthesized, no compounds have found practical use so far. In future research, more useful compounds may be produced from both academic and practical viewpoints. In addition, the synthesis of bicyclo-, spiro-, bicyclic, tricyclic, and tetracyclic dihydropyrimidines should be further studied, despite the many examples that have already been reported for multicyclic dihydropyrimidines. Novel bicyclic and tricyclic dihydropyrimidines may exhibit interesting biological activities and may be more stable than monocyclic dihydropyrimidines, although their bioavailability, metabolism, and toxicology must still be carefully investigated.
This review might be useful for the future synthesis of monocyclic and multicyclic dihydropyrimidines which could be promising compounds from the biological and pharmaceutical viewpoints.
References
1. (a) P. Biginelli, Chem. Ber., 1891, 24, 1317; CrossRef (b) P. Biginelli, Chem. Ber., 1891, 24, 2962; CrossRef (c) P. Biginelli, Gazz. Chim. Ital., 1893, 26, 360.
2. (a) C. O. Kappe, Tetrahedron, 1993, 49, 6937; CrossRef (b) C. O. Kappe and U. G. Wagner, Heterocycles, 1989, 29, 761; CrossRef (c) C. O. Kappe and P. Roschger, J. Heterocycl. Chem., 1989, 26, 55; CrossRef (d) C. O. Kappe, J. Org. Chem., 1997, 62, 7201; CrossRef (e) C. O. Kappe, Eur. J. Med. Chem. 2000, 35, 1043; CrossRef (f) C. O. Kappe and A. Stadler, Org. React. 2004, 63, 1; CrossRef (g) M. Matloobi and C. O. Kappe, J. Comb. Chem., 2007, 9, 275; CrossRef (h) C. O. Kappe, Acc. Chem. Res., 2000, 33, 879; CrossRef (i) A. Lengar and C. O. Kappe, Org. Lett., 2004, 6, 771; CrossRef (j) T. Razzaq, J. M. Kremsner, and C. O. Kappe, J. Org. Chem., 2008, 73, 6321; CrossRef (k) H. Prokopcova and C. O. Kappe, J. Org. Chem., 2007, 72, 4440; CrossRef (l) N. Arshad, J. Hashim, and C. O. Kappe, J. Org. Chem., 2009, 74, 5118; CrossRef (m) C. O. Kappe, G. Uray, P. Roschger, W. Lindner, C. Kratky, and W. Keller, Tetrahedron, 1992, 48, 5473; CrossRef (n) E. Vanden, J. Jean, N. Hecq, O. Kataeva, and C. O. Kappe, Tetrahedron, 2001, 57, 1785; CrossRef (o) H. Prokopcová and C. O. Kappe, Angew. Chem. Int. Ed., 2009, 48, 2276. CrossRef
3. (a) H. Cho, Y. Takeuchi, M. Ueda, and A. Mizuno, Tetrahedron Lett., 1988, 29, 5405; CrossRef (b) H. Cho, M. Ueda, Y. Ohnaka, and M. Hayashimatsu, Heterocycles, 1984, 22, 1959; CrossRef (c) H. Cho, K. Shima, M. Hayashimatsu, Y. Ohnaka, A. Mizuno, and Y. Takeuchi, J. Org. Chem., 1985, 50, 4227; CrossRef (d) H. Cho, Y.Ohnaka, M. Hayashimatsu, M. Ueda, and K. Shima, Tetrahedron Lett., 1986, 27, 6377; CrossRef (e) H. Cho, Y. Hamamoto, K. Shima, and Z. Taira, Tetrahedron, 1986, 42, 6429; CrossRef (f) H. Cho, A. Mizuno, K. Shima, M. Ueda, Y. Takeuchi, M. Hamaguchi, and N. Taniguchi, Heterocycles, 1988, 27, 769; CrossRef (g) H. Cho, T. Iwashita, M. Ueda, A. Mizuno, K. Mizukawa, and M. Hamaguchi, J. Am. Chem. Soc., 1988, 110, 4832; CrossRef (h) H. Cho, M. Ueda, A. Mizuno, T. Ishihara, K. Aisaka, and T. Noguchi, Chem. Pharm. Bull., 1989, 37, 2117; CrossRef (i) H.Cho, M. Ueda, K. Shima, A. Mizuno, M. Hayashimatsu, Y. Ohnaka, Y. Takeuchi, M. Hamaguchi, K. Aisaka, T. Hidaka, M. Kawai, M. Takeda, T. Ishihara, K. Funahashi, F. Satoh, M. Morita, and T. Noguchi, J. Med. Chem., 1989, 32, 2399; CrossRef (j) H. Cho, Y. Nishimura, Y. Yasui, S. Kobayashi, S. Yoshida, E. Kwon, and M. Yamaguchi, Tetrahedron, 2011, 67, 2661; CrossRef (k) H. Cho, Y. Yasui, S. Kobayashi, E. Kwon, M. Arisawa, and M. Yamaguchi, Heterocycles, 2011, 83, 1807; CrossRef (l) H. Cho, E. Kwon, Y. Yasui, S. Kobayashi, S. Yoshida, Y. Nishimura, and M. Yamaguchi, Tetrahedron Lett., 2011, 52, 7185; CrossRef (m) H. Cho, Y. Nishimura, Y. Yasui, and M. Yamaguchi, Tetrahedron Lett., 2012, 53, 1177; CrossRef (n) Y. Nishimura, Y. Yasui, S. Kobayashi, M. Yamaguchi, and H. Cho, Tetrahedron, 2012, 68, 3342. CrossRef
4. (a) B. C. O’Reilly and K. S. Atwal, Heterocycles, 1987, 26, 1185; CrossRef (b) K. S. Atwal, B. C. O’Reilly, J. Z. Gougoutas, and M. F. Malley, Heterocycle, 1987, 26, 1189; CrossRef (c) K. S. Atwal, G. C. Rovnyak, B. C. O’Reilly, and J. Schwartz, J. Org. Chem., 1989, 54, 5898; CrossRef (d) K. S. Atwal, G. C. Rovnyak, J. Schwartz, S. Moreland, A. Hedberg, J. Z. Gougoutas, M. F. Malley, and D. M. Floyd, J. Med. Chem., 1990, 33, 1510; CrossRef (e) K. S. Atwal, G. C. Rovnyak, S. D. Kimball, D. M. Floyd, S. Moreland B. N. Swanson, J. Z. Gougoutas, J. Schwartz, K. M. Smillie, and M. F. Malley, J. Med. Chem., 1990, 33, 2629; CrossRef (f) G. C. Rovnyak, K. S. Atwal, A. Hedberg, S. D. Kimball, S. Moreland, J. Z. Gougoutas, B. C. O’Reilly, J. Schwartz, and M. F. Malley, J. Med. Chem., 1992, 35, 3254; CrossRef (g) K. S. Atwal, B. N. Swanson, S. E. Unger, D. M. Floyd, S. Moreland, A. Hedberg, and B. C. O’Reilly, J. Med. Chem., 1991, 34, 806; CrossRef (h) K. S. Atwal and S. Moreland, Bioorg. Med. Chem. Lett., 1991, 1, 291; CrossRef (i) K. S. Atwal, Eur. Pat. Appl. EP 1988. 254,119; (j) K. S. Atwal, Ger. Offen. DE 1989. 3,839,711.
5. W. Traube and R. Schwarz, Chem. Ber., 1899, 32, 3163. CrossRef
6. S. Ruhemann, J. Chem. Soc, 1903, 83, 1371. CrossRef
7. R. M. Dodson and J. K. Seyler, J. Org. Chem., 1951, 16, 461. CrossRef
8. T. D. Heyes and J. C. Roberts, J. Chem. Soc., 1951, 328. CrossRef
9. E. F. Silversmith, J. Org. Chem., 1962, 27, 4090. CrossRef
10. (a) W. Wendelin and A. Harler, Monatsh. Chem., 1975, 106, 1479; CrossRef (b) W. Wendelin and A. Harler, Monatsh. Chem., 1975, 107, 133; CrossRef (c) W. Wendelin and K. Schermanz, J. Heterocycl. Chem., 1984, 21, 65. CrossRef
11. (a) A. L. Weis and V. P. Mamaev, Izv. Sib. Otd. Akad. Nauk. SSSR. Ser. Khim. Nauk, 1975, 148. (Chem. Abstr., 1976, 84, 121764c); (b) A. L. Weis, Tetrahedron Lett., 1982, 23, 449; CrossRef (c) A. L. Weis, Z. Porat, and Z. Luz, J. Am. Chem. Soc., 1984, 106, 8021; CrossRef (d) A. L. Weis and H. C. van der Plas, Heterocycles, 1986, 24, 1433; CrossRef (e) A. L. Weis and R. Vishkautsan, Heterocycles, 1985, 23, 1077; CrossRef (f) A. L. Weis and R. Vishkautsan, Chem. Lett., 1984, 1773; CrossRef (g) A. L. Weis, F. Frolow, and R. Vishkautsan, J. Org. Chem., 1986, 51, 4623; CrossRef (h) A. L. Weis, Synthesis, 1985, 528; CrossRef (i) A. L. Weis, F. Frolow, D. Zamir, and M. Bernstein, Heterocycles, 1984, 22, 657; CrossRef (j) A. L. Weis and D. Zamir, J. Org. Chem., 1987, 52, 3421; CrossRef (k) A. L. Weis, F. Frolow, and R. Vishkautsan, J. Heterocycl. Chem., 1986, 23, 705; CrossRef (l) A. L. Weis and F. Frolow, J. Org. Chem., 1984, 49, 3635. CrossRef
12. (a) C. Kashima, M. Shimizu, and Y. Omote, Tetrahedron Lett., 1985, 26, 5057; CrossRef (b) C. Kashima, M. Shimizu, and Y. Omote, J. Heterocycl. Chem., 1989, 26, 251; CrossRef (c) C. Kashima, M. Shimizu, A. Tagaya, A. Katoh, and Y. Omote, J. Chem. Res. (S), 1986, 88; (d) A. Katoh, M. Sagane, M. Omote, and C. Kashima, Synthesis, 1983, 409; CrossRef (e) C. Kashima, M. Shimizu, A. Katoh, and Y. Omote, J. Chem. Soc., Perkin Trans. 1, 1983, 1799; CrossRef (f) C. Kashima, M. Shimizu, A. Katoh, and Y. Omote, Tetrahedron Lett., 1983, 24, 209; CrossRef (g) C. Kashima, M. Shimizu, K. Harada, and Y. Omote, J. Heterocycl. Chem., 1988, 25, 1713; CrossRef (h) C. Kashima, M. Shimizu, A. Katoh, and Y. Omote, J. Heterocycl. Chem., 1984, 21, 441; CrossRef (i) C. Kashima, M. Shimizu, and Y. Omote, Chem. Pharm. Bull., 1987, 35, 2694.. CrossRef
13. T. U. Mayer, T. M. Kapoor, S. J. Haggarty, R. W. King, S. L. Schreiber, and T. J. Mitchison, Science, 1999, 286, 971. CrossRef
14. M. A. Ali, E. Manogaran, J. Govindasamy, V. Sellappan, and S. Pandian, J. Enzyme Inhib. Med. Chem., 2011, 26, 149. CrossRef
15. H. Y. K. Kaan, V. Ulaganathan, O. Rath, H. Prokopcova, D. Dallinger, C. O. Kappe, and F. Kozielski, J. Med. Chem., 2010, 53, 5676. CrossRef
16. M. Gartner, N. Sunder-Plassmann, J. Seiler, M. Utz, I. Vernos, T. Surrey, and A. Giannis, ChemBioChem, 2005, 6, 1173. CrossRef
17. N. Caliskan and E. Akbas, Materials Chemistry and Physics, 2011, 126, 983. CrossRef
18. A. R. Katritzky and J. M. Lagowsky, Adv. Heterocycl. Chem., 1963, 1, 311. CrossRef
19. (a) R. E. van der Stoel and H. C. van der Plas, Rec. Trav. Chim., 1978, 97, 116; CrossRef (b) R. E. van der Stoel and H. C. van der Plas, J. Chem. Soc., Perkin Trans. 1, 1979, 1228; CrossRef (c) H. A. J. Holterman, A. van Veldhuizen, and H. C. van der Plas, J. Org. Chem. 1986, 51, 1147. CrossRef
20. W. P. K. Girke, Chem. Ber., 1979, 112, 1. CrossRef
21. J. Milobedzka, St. v. Kostanecki, and V. Lampe, Berichte der Deutschen Chemischen Gesellshaft, 1910, 43, 2163. CrossRef
22. A. Mobinikhaledi, N. Forughifar, J. A. Safari, and E. Amini, J. Heterocycl. Chem., 2007, 44, 697. CrossRef
23. S. Hoffmann and E. Muehle, Z. Chem., 1969, 9, 66.
24. B. C. Merja, A. M. Joshi, K. A. Parikh, and A. R. Parikh, Indian J. Chem., Section B, 2004, 43, 909.
25. K. Rana, B. Kaur, and B. Kumar, Indian J. Chem., Section B, 2004, 43, 1553.
26. E. E. Wyatt, W. R. J. D. Galloway, G. L. Thomas, D. R. Spring, M. Welch, O. Loiseleur, and A. T. Plowright, Chem. Commun., 2008, 4962. CrossRef
27. B. Han, R.-F. Han, Y.-W. Ren, X.-Y. Duan, Y.-C. Xu, and W. Zhang, Tetrahedron, 2011, 67, 5615. CrossRef
28. M. M. F. Ismail, N. A. M. El-Sayed, H. S. Rateb, M. Ellithey, and Y. A. Ammar, Arzneimittelforschung, 2006, 56, 322.
29. C. A. Sehon, G. Z. Wang, A. Q. Viet, K. B. Goodman, S. E. Dowdell, D. Lee, R. Bentley, C. P. Doe, D. J. Behm, E. Hu, C. Evans, L. J. Jolivette, P. A. Elkins, S. F. Semus, R. B. Kirkpatrick, E. Dul, S. S. Khandekar, T. Yi, L. L. Wright, and G. K. Smith, J. Med. Chem., 2008, 51, 6631. CrossRef
30. B. Sain, S. P. Singh, and J. S. Sandhu, Tetrahedron, 1992, 48, 4567. CrossRef
31. (a) S. N. Mazumdar, S. Mukherjee, A. K. Sharma, D. Sengupta, and M. P. Mahajan, Tetrahedron, 1994, 50, 7579; CrossRef (b) S. Jayakumar, P. Singh, and M. P. Mahajan, Tetrahedron, 2004, 60, 4315; CrossRef (c) A. K. Sharma and M. P. Mahajan, Tetrahedron, 1997, 53, 13841; CrossRef (d) C. Mohan, P. Singh, and M. P. Mahajan, Tetrahedron, 2005, 61, 10774. CrossRef
32. I. Ibnusaud, E. J. P. Malar, and N. Sundaram, Tetrahedron Lett., 1990, 31, 7357. CrossRef
33. A. Guzman, M. Romero, F. X. Talamas, R. Villena, R. Greenhouse, and J. M. Muchowski, J. Org. Chem., 1996, 61, 2470. CrossRef
34. R. S. Shadbolt and T. L. V. Ulbricht, J. Chem. Soc. C, 1968, 6, 733. CrossRef
35. S. Baskaran, E. Hanan, D. Byun, and W. Shen, Tetrahedron Lett., 2004, 45, 2107. CrossRef
36. B. K. Singh, M. Mishra, N. Saxena, R. P. Tripathi, G. P. Yadav, P. R. Maulik, M. K. Sahoo, R. L. Gaur, and P. K. Murthy, Eur. J. Med. Chem., 2008, 43, 2717. CrossRef
37. S. Singh, A. Schober, M. Gebinoga, and G. G. Alexander, Tetrahedron Lett., 2009, 50, 1838. CrossRef
38. D. A. Ibrahim and A. M. El-Metwally, Eur. J. Med. Chem., 2010, 45, 1158. CrossRef
39. O. Alam, S. A. Khan, N. Siddiqui, W. Ahsan, S. P. Verma, and S. J. Gilani, Eur. J. Med. Chem., 2010, 45, 5113. CrossRef
40. N. N. Karade, V. S. Gampawar, N. P. Tale, and S. B. Kedar, J. Heterocycl. Chem., 2010, 47, 740. CrossRef
41. R. A. Baxter and F. S. Spring, J. Chem. Soc., 1947, 1179. CrossRef
42. (a) K. Folkers and T. B. Johnson, J. Am. Chem. Soc., 1933, 55, 2886; CrossRef (b) K. Folkers and T. B. Johnson, J. Am. Chem. Soc., 1934, 56, 1374. CrossRef
43. E. L. Khanina, E. E. Liepin’sh, D. Kh. Mutsenietee, and G. Duburs, Khim. Geterotsikl. Soed., 1987, 668.
44. R. F. Doehner, Abstracts of Papers, Tenth International Congress of Heterocyclic Chemistry, Ontario, Canada, 1985, S 5-31.
45. A. J. Zych, H.-J. Wang, and S. A. Sakwa, Tetrahedron Lett., 2010, 51, 5103. CrossRef
46. G. Zigeuner, C. Knopp, and H. Blaschke, Monatsh. Chem., 1976, 107, 587. CrossRef
47. T. George, R. Tahilramani, and D. V. Metha, Synthesis, 1975, 405. CrossRef
48. M. S. Akhtar, M. Seth, and A. P. Bhaduri, Indian J. Chem., 1987, 26B, 556.
49. K. Yamamoto, Y. G. Chen, and F. G. Buono, Org. Lett., 2005, 21, 4673. CrossRef
50. Y. Yamamoto, A. Sakaguchi, H. Yoshida, and K. Akiba, J. Chem. Soc., Perkin Trans. 1, 1988, 725. CrossRef
51. K. Ozeki, T. Ichikawa, H. Takehara, K. Tanimura, M. Sato, and H. Yaginuma, Chem. Pharm. Bull., 1989, 37, 1780. CrossRef
52. S. Seto and Y. Kohno, Heterocycles, 2009, 78, 2263. CrossRef
53. J. Spychała, Synth. Commun., 1997, 27, 1943. CrossRef
54. R. Waelchli, B. Bollbuck, C. Bruns, T. Buhl, J. Eder, R. Feifel, R. Hersperger, P. Janser, L. Revesz, H.-G. Zerwes, and A. Schlapbach, Bioorg. Med. Chem. Lett., 2006, 16, 108. CrossRef
55. W. Wendelin and K. J. Schermanz, J. Heterocycl. Chem., 1984, 21, 65. CrossRef
56. (a) S. K. Collins, A. I. McDonald, L. E. Overman, and Y. H. Rhee, Org. Lett., 2004, 6, 1253; CrossRef (b) B. L. Nilsson and L. E. Overman, J. Org. Chem., 2006, 71, 7706; CrossRef (c) F. Cohen and L. E. Overman, J. Am. Chem. Soc., 2006, 128, 2594; CrossRef (d) F. Cohen and L. E. Overman, J. Am. Chem. Soc., 2006, 128, 2604; CrossRef (e) F. Cohen, S. K. Collins, and L. E. Overman, Org. Lett., 2003, 5, 4485; CrossRef (f) C. A. Bowley, S. Ray, F. Cohen, S. K. Collins, and L. E. Overman, J. Nat. Prod., 2004, 67, 1319. CrossRef
57. F. Acquadro, H. Oulyadi, P. Venturello, and J. Maddaluno, Tetrahedron Lett., 2002, 43, 8759. CrossRef
58. Y. Tamura, N. Tsujimoto, and M. Uchimura, Chem. Pharm. Bull., 1971, 19, 143. CrossRef
59. (a) R. E. van der Stoel and H. C. van der Plas, J. Chem. Soc., Perkin Trans. 1, 1979, 1228; CrossRef (b) R. E. van der Stoel and H. C. van der Plas, J. Chem. Soc., Perkin Trans. 1, 1979, 2393. CrossRef
60. (a) J. Ashby and D. Griffiths, J. Chem. Soc., Perkin Trans. 1, 1975, 657; CrossRef (b) J. Ashby and D. Griffiths, J. Chem. Soc., Chem. Commun., 1974, 607. CrossRef
61. E. Bullock, R. A. Carter, R. M. Cochrane, B. Gregory, and D. C. Shields, Can. J. Chem., 1977, 55, 895. CrossRef
62. E. Bullock, R. A. Carter, B. Gregory, and D. C. Shields, J. Chem. Soc., Chem. Commun.,1972, 97. CrossRef
63. D. A. Claremon and S. A. Rosenthal, Synthesis, 1986, 664. CrossRef
64. J. J. Baldwin, D. E. McClure, and D. A. Claremon, US Patent 4677102, 1987.
65. G. Zigeuner and C. Knopp, Monatsh. Chem., 1970, 101, 1541. CrossRef
66. (a) B. B. Snider and Z. Shi, Tetrahedron Lett., 1993, 34, 2099; CrossRef (b) B. B. Snider and Z. Shi, J. Am. Chem. Soc., 1994, 116, 549. CrossRef
67. D. Bozing, P. Benko, L. Petocz, M. Szecsey, P. Toempe, G. Gigler, I. Gacsalyi, and I. Gyertyan, (EGIS Gyogyszergyar) Eur. Pat. Appl. EP 1991, 409,233.
68. F. Aslanoglu, E. Akbas, M. Soenmez, and B. Anil, Phosphorus, Sulfur Silicon Relat. Elem., 2007, 182, 1589. CrossRef
69. A. Dandia, S. Khaturia, P. Sarawgi, and A. Jain, Phosphorus, Sulfur Silicon Relat. Elem., 2007, 182, 2529. CrossRef
70. E. E. Wyatt, S. Fergus, W. R. J. D. Galloway, A. Bender, D. J. Fox, A. T. Plowright, A. S. Jessiman, M. Welch, and D. R. Spring, Chem. Comm., 2006, 3296. CrossRef
71. V. Sangeetha and K. J. R. Prasad, Indian J. Chem., Section B, 2006, 45, 1028.
72. A. Y. Potapov, K. S. Shikhaliev, D. V. Krylsky, and M. Y. Krisin, Khim. Geterotsikl. Soed.., 2006, 42, 1549 (Chem. Heterocycl. Compd., 2006, 42, 1338). CrossRef
73. Bayer A.G. patent, DE 3212737.5, 1982.
74. D. W. McKinstry and E. H. Reading, J. Franklin. Inst., 1944, 237, 422. CrossRef
75. Nizamuddin, M. Mishra, M. K. Srivastava, and M. H. Khan, Indian J. Chem., Section B, 2001, 40, 49.
76. H. J. M. Gijsen, D. Berthelot, M. A. J. De Cleyn, I. Geuens, B. Brône, and M. Mercken, Bioorg. Med. Chem. Lett., 2012, 22, 797. CrossRef
77. S. G. Duron and D. Y. Gin, Org. Lett., 2001, 3, 1551. CrossRef
78. K. Cooper (Pfizer Ltd.) PCT Int. Appl. WO 1990, 11,281.
79. D. M. Stout and A. I. Meyers, Chem. Rev., 1982, 82, 223. CrossRef
80. M. Tisler and B. Stanovnik, Adv. Heterocycl. Chem., 1968, 9, 211. CrossRef
81. Bayer A.G. patent, DE 834624, 1978.
82. R. R. Shimidt, Angew. Chem., Int. Ed. Engl., 1975, 14, 581. CrossRef
83. J.-L. Fourrey, J. Beauhaire, and C. W. Yuan, J. Chem. Soc., Perkin Trans. 1, 1987, 1841. CrossRef
84. Bayer A.G. patent, DE 3400765, 1984.
85. Bayer A.G. patent, DE 2235406.9, 1972.
86. E. W. Hurst and R. Hull, J. Med. Pharm. Chem., 1961, 3, 215. CrossRef
87. T. Kato, Japan Kokai Tokkyo Koho, JP 1984, 59,190,974.
88. Y. S. Sadanandam, M. M. Shetty, and P. V. Diwan, Eur. J. Med. Chem., 1992, 27, 87. CrossRef
89. E. L. Khanina, G. Siliniece, J. Ozols, G. Duburs, and A. Kimenis, Khim.-Farm. Zh., 1978, 12, 72.
90. T. U. Mayer, T. M. Kapoor, S. J. Haggarty, R. W. King, S. L. Schreiber, and T. J. Mitchison, Science, 1999, 286, 971. CrossRef
91. S. Gore, S. Baskaran, and B. Koenig, Green Chem., 2011, 13, 1009. CrossRef
92. C. D. Cox, M. J. Breslin, B. J. Mariano, P. J. Coleman, C. A. Buser, E. S. Walsh, K. Hamilton, H. E. Huber, N. E. Kohl, M. Torrent, Y. Yan, L. C. Kuo, and G. D. Hartman, Bioorg. Med. Chem. Lett., 2005, 15, 2041. CrossRef
93. M. A. Ali, E. Manogaran, J. Govindasamy, V. Sellappan, and S. Pandian, J. Enzyme Inhib. Med. Chem., 2011, 26, 149. CrossRef
94. E. E. Wyatt, W. R. J. D. Galloway, G. L. Thomas, D. R. Spring, M. Welch, O. Loiseleur, and A. T. Plowright, Chem. Commun., 2008, 4962. CrossRef
95. C. A. Sehon, G. Z. Wang, A. Q. Viet, K. B. Goodman, S. E. Dowdell, D. Lee, R. Bentley, C. P. Doe, D. J. Behm, E. Hu, C. Evans, L. J. Jolivette, P. A. Elkins, S. F. Semus, R. B. Kirkpatrick, E. Dul, S. S. Khandekar, T. Yi, L. L. Wright, and G. K. Smith, J. Med. Chem., 2008, 51, 6631. CrossRef
96. X.-C. Wang, Y. Wei, Y.-X. Da, Z. Zhang, and Z.-J. Quan, Heterocycles, 2011, 83, 2811. CrossRef
97. R. V. Chikhale, R. P. Bhole, P. B. Khedekar, and K. P. Bhusari, Eur. J. Med. Chem., 2009, 44, 3645. CrossRef
98. M. Dabiri, S. Cobra Azimi, H. Arvin-Nezhad, and A. Bazgir, Heterocycles, 2008, 75, 87. CrossRef
99. X. Zhu, X. Zhou, X. Yang, G. Zhao, X. Xu, G. Xia, Z. Zheng, L. Wang, and S. Li, Bioorg. Med. Chem. Lett., 2010, 20, 299. CrossRef
100. K. Arya and A. Dandia, J. Fluor. Chem., 2007, 128, 224. CrossRef
101. M. S. K. Youssef and A. A. Omar, Monatsh. Chem., 2007, 138, 989. CrossRef
102. P. K. Sarvesh and K. Nizamuddin, Arch. Pharm. (Weinheim), 2008, 341, 418. CrossRef
103. M. Yu, S. S. Pochapsky, and B. B. Snider, J. Org. Chem., 2008, 73, 9065. CrossRef
104. T. Mishina, N. Tsuda, A. Inui, and Y. Miura, Jpn. Kokai Tokkyo Koho, JP 1987, 62,169,793.
105. C. Landreau, D. Deniaud, A. Reliquet, and J. C. Meslin, Eur. J. Org. Chem., 2003, 3, 421. CrossRef
106. D. Bozing, P. Benko, L. Petocz, M. Szecsey, P. Toempe, G. Gigler, I. Gacsalyi, and I. Gyertyan, Eur. Pat. Appl. EP 1991, 409,233.
107. A. Balkan, M. Ertan, and T. Burgemeister, Arch. Pharm. (Weinheim), 1992, 325, 499. CrossRef
108. S. D. Sharma, V. Kaur, P. Bhutani, and J. P. S. Khurana, Bull. Chem. Soc. Jpn., 1992, 65, 2246. CrossRef